the Creative Commons Attribution 4.0 License.
the Creative Commons Attribution 4.0 License.
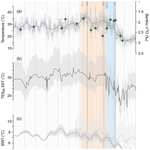
Middle Miocene climate evolution in the northern Mediterranean region (Digne–Valensole basin, SE France)
Armelle Ballian
Maud J. M. Meijers
Isabelle Cojan
Damien Huyghe
Miguel Bernecker
Katharina Methner
Mattia Tagliavento
Jens Fiebig
Andreas Mulch
During the Middle Miocene, the Earth shifted from a warm state, the Miocene Climatic Optimum (MCO; 16.9–14.7 Ma), to a colder state associated with the formation of extensive and permanent ice sheets on Antarctica. This climatic shift, the Middle Miocene Climatic Transition (MMCT; 14.7–13.8 Ma) strongly affected the composition and structure of major biomes, ocean circulation, and precipitation patterns. Although Middle Miocene climate dynamics are well documented in marine records, our knowledge of terrestrial climate change is not well constrained. Here we present a long-term (23–13 Ma) stable (δ13C, δ18O) and clumped (Δ47) isotope record of soil carbonates from a northern Mediterranean Alpine foreland basin: the Digne–Valensole basin (DVB), France. Δ47-derived soil carbonate formation temperatures indicate a highly dynamic dry season temperature pattern that is consistent with multiple periods of reorganization of atmospheric circulation during the MCO. We propose that changes in atmospheric circulation patterns modified the seasonality of precipitation and, ultimately, the timing of pedogenic carbonate formation. Consequently, Δ47 soil carbonate temperature data record the combined effects of long-term regional temperature and carbonate formation seasonality change. The data are consistent with the existence of a proto-Mediterranean climate already during certain MCO time intervals. Following the MMCT, the stable and clumped isotope record displays pronounced cooling after 13.8 Ma accompanied by a rather large (−5.0 %) decrease in soil water δ18O values. Our northern Mediterranean foreland basin climate record shares strong similarities with time-equivalent records from the terrestrial European mid-latitudes and the global oceans and enhances our understanding of the circum-Alpine Middle Miocene terrestrial climate dynamics.
- Article
(5965 KB) - Full-text XML
-
Supplement
(6385 KB) - BibTeX
- EndNote
The Miocene Climatic Optimum (MCO; 16.9–14.7 Ma) represents a warm but dynamic climatic interval and was followed by a transition to a colder state, the Middle Miocene Climatic Transition (MMCT; 14.7–13.8 Ma). The MMCT was associated with the inception of major, permanent Antarctic ice sheets (e.g., Flower and Kennett, 1994; Holbourn et al., 2005) and is considered to prelude one of the major cooling steps in Earth's Cenozoic climate history (Flower and Kennett, 1994; Holbourn et al., 2007). Global cooling had profound impacts on the distribution of major biomes (Jiménez-Moreno and Suc, 2007), global ocean circulation and ice sheet dynamics (e.g., Holbourn et al., 2014; Zachos et al., 2001), and continental temperature and precipitation patterns (e.g., Methner et al., 2020; Pound et al., 2012).
The MCO corresponds to one of the warmest intervals of the Neogene period (Flower and Kennett, 1994; Holbourn et al., 2022; Zachos et al., 2001). With higher global temperatures and moderately higher atmospheric CO2 concentrations (; Steinthorsdottir et al., 2021a) than today, it bears characteristics of future global warming scenarios (Longman et al., 2022; Steinthorsdottir et al., 2021b). Refining the understanding of climate variability during the MCO may, therefore, underpin projections of anthropogenic climate change, particularly for the (circum-)Mediterranean region, which has been suggested to become highly vulnerable to intensified future droughts (e.g., Lionello, 2012).
Despite the intense study of Earth's Miocene climate history, our knowledge of Middle Miocene continental climate dynamics remains fragmentary. Efforts in reconstructing European continental climate conditions during the Middle Miocene focused on central (e.g., Jiménez-Moreno et al., 2008; Methner et al., 2020; Mosbrugger et al., 2005) or southern Europe (e.g., Abels et al., 2009; Jiménez-Moreno and Suc, 2007) and highlight possible latitudinal differences in (hydro-)climate (Fauquette et al., 2007; Jiménez-Moreno and Suc, 2007).
Well-dated paleosol sequences provide excellent archives to study long-term continental (hydro-)climatic changes (e.g., Quade et al., 2007), soil formation (Sheldon and Tabor, 2009a), and soil water characteristics within soil profiles (Zamanian et al., 2021). They also allow us to estimate climate parameters such as mean annual precipitation (MAP), mean annual temperature (MAT) (Gillot et al., 2022; Retallack, 2005), and soil temperatures during pedogenic carbonate formation through clumped isotope thermometry (e.g., Kelson et al., 2018; Meijer et al., 2024; Methner et al., 2020). Clumped isotope (Δ47) thermometry allows us to reconstruct the formation temperature of (pedogenic) carbonate, independent of the oxygen isotopic composition of the parental fluid (Eiler, 2011). By simultaneously obtaining carbon (δ13C) and oxygen (δ18O) isotopic compositions in addition to Δ47 values of pedogenic carbonates, it is possible to constrain the oxygen isotopic composition of soil water (δ18Ow) assuming equilibrium fractionation (Kim and O'Neil, 1997). This approach was successfully applied to paleosols to document long-term continental temperature change, temperature seasonality, and changes in moisture sources (e.g., Kelson et al., 2018; Meijer et al., 2024; Methner et al., 2020; Rugenstein et al., 2022). Embedded within a solid geochronological framework, such paleosol records in terrestrial basins may hence provide continental paleoclimatic information with a temporal resolution that permits direct comparison with marine proxy records (e.g., Methner et al., 2020).
Here, we present δ18O, δ13C, and Δ47 data from pedogenic carbonate nodules of the Digne–Valensole basin (DVB; France, northern Mediterranean region) from ca. 23–13 Ma. Today, the DVB is located at an intermediate latitude between the well-studied Middle Miocene localities in southern (Madrid and Calatayud–Daroca basins, Spain; e.g., Abels et al., 2009) and central (e.g., North Alpine Foreland Basin (NAFB), Switzerland; Kälin and Kempf, 2009; Methner et al., 2020) Europe.
2.1 Geology of the Digne–Valensole basin
At the intersection of Mediterranean, semi-continental, and mountainous climates, the modern DVB – as part of the western Alpine foreland basin – is characterized by an altered Mediterranean climate (“climat méditerranéen altéré”; Joly et al., 2010). The latter differs from the typical Mediterranean climate by a higher frequency of “cold” days and a greater annual precipitation amount (800–950 mm) distributed non-uniformly across the year (Joly et al., 2010).
Mediterranean climate is defined as semi-arid, with dry and hot summers that contrast mild and wet winters. During the winter months, cool and wet air masses from the North Atlantic Ocean deliver moisture to the western Alpine foreland, including the DVB (e.g., Seager et al., 2019). During the summer months, a shift in prevailing wind patterns leads to progressively drier conditions. Moisture from the Mediterranean Sea is episodically transported northward to the DVB region by southerly–southwesterly winds. The Eocene to Pliocene Digne–Valensole basin represents a deformed segment of the Alpine foreland basin system that extends along a narrow SW–NE-trending belt west of the Western Alps (Fig. 1a and b). The DVB molasse currently covers ca. 1500 km2 (Fig. 1a) and is bordered by the Digne thrust front in the north and east and the Durance strike-slip fault in the west (Fig. 1b; Ford et al., 1999).
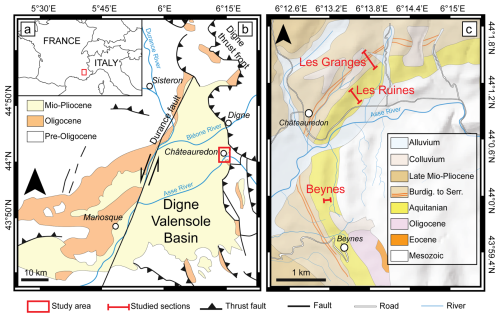
Figure 1(a) Map showing southern central Europe. The location of the Digne–Valensole basin (DVB) in SE France is indicated by the red rectangle. (b) Geological map of the DVB and study area (red rectangle) at the margin of the Digne thrust front (after Gillot et al., 2022). (c) Locations of the Les Granges, Les Ruines, and Beynes sections within the DVB (after Cojan and Gillot, 2022).
Combined advancement of the Alpine orogenic front and variations in sea level (Bauer, 2006; Bialkowski, 2002; Cojan et al., 2013) affected the Late Eocene to Pliocene basin fill. The overall ca. 3000 m thick Tertiary basin succession (the “Digne Molasse”; Cojan et al., 2013, and references therein; Gigot et al., 1974) is composed of alternating continental to shallow marine deposits with lateral variations in facies and stratigraphic thickness and internal unconformities (Gigot et al., 1974). The Digne Molasse (Gigot et al., 1974) is divided into four formations, which include, from base to top, (1) the Chattian Molasse Rouge (thickness of up to 500 m), which is characterized by alluvial and fluvial facies; (2) the Chattian–Burdigalian Molasse Grise or Molasse Intermédiaire (thickness of several hundreds of meters), which consists of deltaic and distal floodplain deposits; (3) the Burdigalian–Tortonian Molasse Marine (thickness up to 1600 m), dominated by tidal and coastal deposits and characterized by several transgression–regression cycles; and (4) the Miocene–Pliocene Molasse Jaune (thickness up to 300 m), made up of coarse-grained alluvial sedimentary rocks. Most of the material in this study originates from soil horizons within the Molasse Grise and Molasse Marine formations, along with a few samples from the Molasse Rouge formation.
2.2 Middle Miocene climate variability
The Miocene (23.03–5.33 Ma) period is characterized by major environmental change, including cool periods associated with the glaciation of Antarctica and the Northern Hemisphere that were interrupted by warm events, such as the Miocene Climatic Optimum (MCO; 16.9–14.7 Ma; Holbourn et al., 2014, 2015; Jiménez-Moreno et al., 2005; Mudelsee et al., 2014). Well-dated oceanic records provide numerous records of Miocene climate (e.g., Flower and Kennett, 1994; Holbourn et al., 2014, 2015; Mudelsee et al., 2014; Westerhold et al., 2020; Zachos et al., 2001). Following protracted Cenozoic cooling, a stepwise trend of increasing temperatures accompanied by short-term cooling events is documented from megafloras for the Early Miocene in central Europe (Early Aquitanian; Mosbrugger et al., 2005). The MCO reflects the warmest episode of the Neogene with minimum global ice volume (Holbourn et al., 2007, 2014, 2015; Jiménez-Moreno and Suc, 2007; Mudelsee et al., 2014; Steinthorsdottir et al., 2021b). The drivers of MCO warming and its geographic and temporal variability during the MCO are still debated. The onset of the MCO coincides with the 17–16 Ma Columbia River Basalt eruptions in western North America (Longman et al., 2022; Steinthorsdottir et al., 2021b). Combined with increased fluxes of CO2 in the atmosphere caused by volcanic arc or rift activity and decreased continental weatherability, the Columbia River Basalt eruptions may have triggered the transition into the warm MCO (Longman et al., 2022, and references therein). Although the MCO was generally an interval of intense warmth, high-resolution benthic foraminiferal δ18O records also indicate high-amplitude climate variability, particularly towards its end (15.7–14.7 Ma; Holbourn et al., 2007, 2014, 2022).
While the mid-latitude N–S temperature gradient in western Europe was probably reduced during the Middle Miocene (Jiménez-Moreno and Suc, 2007), continental paleoclimate reconstructions of the MCO emphasize a strong semi-arid to humid latitudinal gradient with annual precipitation amounts lower than today (i.e., herpetological data from Böhme, 2004; mammal diversity from Costeur and Legendre, 2008; pollen records from Jiménez-Moreno and Suc, 2007). Southern mid-latitude locations, such as southern central Spain, likely experienced semi-arid climatic conditions with open environments (subdesertic conditions; Urban et al., 2010), whereas more northern regions, e.g., central eastern France and Switzerland, were characterized by a more humid climate (1000–1500 mm yr−1; Böhme, 2004) and pronounced precipitation seasonality (Botsyun et al., 2022; Costeur and Legendre, 2008, and references therein). The existence of a Middle Miocene latitudinal aridity gradient stretching from southern to northern mid-latitudinal Europe may have resulted from a trade wind system, which was characterized by prevalent northeasterly winds. Orography may have contributed to the zonal precipitation pattern: trade winds produced summer precipitation upwind and dryness downwind of the east–west-oriented European orogens (Pyrenees and Alps; Böhme, 2004). The demarcation between westerlies and trade winds was likely positioned north of 47° N paleolatitude (Böhme, 2004). Paleoclimate modeling suggests this pattern had ceased by the Late Miocene with a shift in European atmospheric circulation towards westerly dominated winds (Quan et al., 2014).
After the MCO, significant cooling led to the renewed expansion of the Antarctic ice sheet and deep-water cooling during the MMCT (14.7–13.8 Ma; Holbourn et al., 2014; Miller et al., 1991). The latter had a profound impact on marine and terrestrial realms, e.g., the global reorganization of ocean circulation patterns (Holbourn et al., 2022), the increase in foraminifera taxa, the decline in crustacean diversity (Steinthorsdottir et al., 2021b, and references therein), the gradual disappearance of subdesertic plants in southwestern Europe (Jiménez-Moreno and Suc, 2007), and the extinction of central European alligators and giant turtles (Böhme, 2004). The MMCT also impacted terrestrial temperature and precipitation patterns with enhanced temperature seasonality and a shift towards a westerly dominated wind regime over central Europe by the Tortonian (Methner et al., 2020; Quan et al., 2014).
2.3 Present-day Mediterranean climate
The main characteristics of the present-day climate in the circum-Mediterranean region – a marked seasonality between mild, maritime, wet winters and warm to hot, dry summers (Lionello, 2012; Seager et al., 2019) – have been recorded as early as the Pliocene (Suc, 1984). The timing of the onset of the characteristic marked seasonality is, however, still unclear. Typically, North Atlantic storm tracks deliver precipitation to the region during the winter months. Below-freezing temperatures rarely occur during these months, except in mountainous areas. During the summer season, mid-latitude storm tracks weaken due to the dominance of the Azores High pressure system, resulting in warm to hot and dry summers (Seager et al., 2019; Ulbrich et al., 2012).
Being influenced by both a mid-latitude climate variability steered by the North Atlantic Oscillation (NAO) and subtropical conditions due to the descending branch of the Hadley cell, the overall temperature and precipitation patterns in the circum-Mediterranean region reflect the interaction of many individual drivers, including orography, latitude, and land–sea distribution (Ulbrich et al., 2012).
3.1 Pedogenic carbonate horizons and sampling strategy
Stratigraphic correlations between the investigated sections permit the construction of a ca. 500 m long composite section (Châteauredon composite section; BCR) that consists of three correlated sections: Beynes (BE), Ruines (RU), and Les Granges (GR; Fig. 1c; Bialkowski et al., 2006; Cojan et al., 2013; Gillot et al., 2022). Based on bio- and δ13C chemostratigraphy, the BCR covers the Early to Middle Miocene from ca. 23 to ca. 13 Ma (Bialkowski et al., 2006; Cojan et al., 2013). Due to its proximity to the Miocene shoreline, the stratigraphy of the BCR consists of alluvial, lacustrine, fluvial, marine, and coastal facies with intercalations of continental deposits including numerous paleosol horizons. More than 90 % of the paleosol horizons can be classified as calcisols with irregularly shaped and globular, hard micritic pedogenic carbonate nodules dispersed in a fine-grained mudstone, shale, or siltstone matrix (Cojan and Gillot, 2022). The pedogenic carbonate nodules formed on floodplain alluvium and are found in isolated accumulation (Bk) horizons. The nodules range from a few millimeters to several centimeters in diameter and are commonly spheroidal with irregularities (see Fig. 2c).
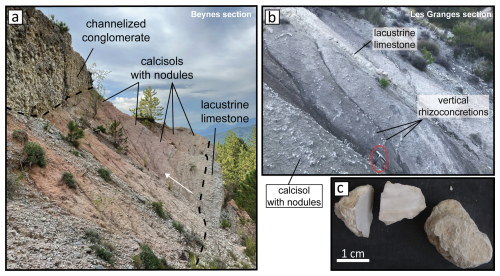
Figure 2Field photographs. (a) Beynes section: paleosols that developed in distal alluvial fan deposits (channelized conglomerates; modified after Cojan and Gillot, 2022). The white arrow indicates the younging direction of stratigraphy orientation. (b) Les Granges section: stacked horizons of pedogenic carbonate-rich paleosols that contain vertical rhizoconcretions and are overlain by lacustrine limestone. Hammer for scale. (c) Pedogenic carbonate nodules from the Les Granges section.
In this study, 16 pedogenic carbonate samples from different Bk horizons within the BCR were analyzed for δ13C, δ18O, and Δ47 paleothermometry. Individual pedogenic carbonate nodules are from Bk horizons of carbonate-rich mature paleosols and correspond to the sampling horizons of Bialkowski (2002) and complement the existing δ13C record (Bialkowski et al., 2006; Figs. 2 and S1 in the Supplement). Nodules were sampled 50–100 cm below the preserved soil top, which at times corresponds to a truncation surface. Therefore, the formation depth of the carbonate nodules was at least 50 cm (Bialkowski, 2002). Two pedogenic carbonate nodules come from the fine-grained alluvial succession of the Beynes section, which is dominated by pink to reddish marls of the Molasse Rouge. A total of 14 pedogenic carbonate nodules originate from the transgression–regression cycles of the Molasse Grise and the Marine Formation of the Ruines and Granges sections (Table 1 and Fig. S3 in the Supplement).
3.2 Age model
The initial age model for the BCR composite section relies on integrated biostratigraphy and chemostratigraphy (δ13C values of pedogenic carbonate; Bialkowski et al., 2006; Cojan et al., 2013). The age model is based on the correlation of trends in pedogenic carbonate δ13C values (n=76) with trends in marine reference δ13C records (compilation of ODP–DSDP sites; Bialkowski et al., 2006, and references therein). Each correlated time interval of the BCR section was validated by biostratigraphy (micromammal faunas and gastropod fossils) and palynological analysis (dinocysts, spores, and pollen, including a distinct Avicennia peak; Châteauneuf et al., 2006). The initial age model adopted the timescale of Berggren et al. (1995).
Here, we adopt and update the initial age model by tying the δ13C curve of the BCR composite section to the more recent benthic deep-sea foraminifera carbon isotope curve from Westerhold et al. (2020) by using the QAnalySeries software (Kotov and Paelike, 2018). We use the same tie points (e.g., positive or negative δ13C excursions) between the continental and reference marine records as Bialkowski et al. (2006) and Cojan et al. (2013). For intervals with limited tie points and matching trends, the correlation was based on the best fit provided by the software assuming uniform sedimentation rates and ensuring similar trends with the reference marine δ13C curve (Fig. S4; Tables S2 and S3 in the Supplement).
Age uncertainties in the DVB range from 100 ka to 1 Ma (Fig. 3; Bialkowski, 2002). The age uncertainties in the DVB are constrained by the correlation of the DVB biostratigraphy with the biostratigraphy of the well-correlated magnetostratigraphic NAFB and the well-described biostratigraphy (MN zones) of the Aquitaine basin (Bialkowski, 2002). Given that individual carbonate nodules in the paleosols may form over hundreds to thousands of years (Gile et al., 1966; Kelson et al., 2020), the uncertainty in the age model is larger than the time integrated by the individual carbonate nodules.
3.3 Carbonate stable isotope analysis and Δ47 thermometry
Clumped isotope thermometry (Δ47) relies on the determination of the temperature-dependent excess abundance of rare carbon and oxygen isotope bonds (13C–18O), the so-called clumping, within the carbonate crystal lattice. The isotopic clumping of carbonate molecules is dependent on the temperature of carbonate formation but independent of the δ18O value of the fluid from which the carbonate precipitated (Ghosh et al., 2006; Eiler, 2011).
Isotope analyses were performed at the Goethe University–Senckenberg BiK-F Joint Stable Isotope Facility (Frankfurt, Germany). Measurements were performed on a Kiel IV Carbonate Device coupled to an MAT 253 Plus IRMS. For a detailed method description, refer to the Supplement. Prior to measurements, carbonate nodules were cut and subsequently powdered with a dental drill.
Samples were analyzed in a variable number of replicates () using between 90 and 190 µg of material according to carbonate content. Samples were analyzed together with the ETH-1–ETH-4 carbonate standards (Bernasconi et al., 2018). Raw intensity data were corrected for the negative pressure baseline using the signal and scaling factor method (Bernecker et al., 2023; Fiebig et al., 2021) applied to ETH-1 and ETH-2. Standardization was carried out by utilizing the D47crunch module (Daëron, 2021) in pooled sessions mode with ETH standards as carbonate anchors (Δ47 (I-CDES), Bernasconi et al., 2021). The universal temperature calibration of Anderson et al. (2021) is used for temperature conversion.
Stable isotope results of pedogenic carbonates are normalized relative to nominal values of ETH standards (Bernasconi et al., 2018) and reported in per mille deviation relative to V-PDB (δ13Ccarb, δ18Ocarb). δ18Ow was calculated based on our obtained carbonate formation temperature (T(Δ47)) and δ18Ocarb data and on the oxygen isotope equilibrium fractionation equation of Kim and O'Neil (1997). δ13Ccarb and δ18Ocarb errors are reported as 1 standard deviation ( ±1 SD; see tables in the Supplement), whereas Δ47 errors are reported as 1 standard deviation, fully propagated 1 standard error (1 SE), and fully propagated 2 standard error (2 SE; Tables 1 and S1 in the Supplement).
Complete results of stable and clumped isotope measurements of the pedogenic carbonate nodules are presented in Table S1. The analyses of pedogenic carbonate nodules of the same horizon (see Methodology) complement data presented in Bialkowski (2002) (Table 1). The following sections discuss the results from analyses carried out in this study.
4.1 Stable (δ18Ocarb and δ13Ccarb) and clumped (Δ47) isotope data
δ18Ocarb values of paleosol carbonate samples from the Digne–Valensole basin range from −7.02 ‰ to −4.66 ‰ (V-PDB), and δ13Ccarb values range from −9.90 ‰ to −6.15 ‰ (V-PDB). The Δ47 (I-CDES) values of pedogenic carbonate range from 0.565 ‰ to 0.619 ‰, which translates to a temperature range of 35.2–16.8 °C. External standard errors (1 SE) for 8–18 replicate measurements range from 0.01 ‰ to 0.02 ‰, which corresponds to temperature uncertainties covering 3.5–6.0 °C. Calculated soil water δ18Ow values based on corresponding δ18Ocarb and Δ47 values range from −6.29 ‰ to −0.51 ‰ (V-SMOW).
Due to potential diagenetic alteration, measurements from one sample (98GR22) are not included in the interpretation of the results and the Discussion (Table S1 and Fig. S2 in the Supplement). This sample yields a clumped isotope temperature (T(Δ47)=39.2 °C), which is slightly higher than plausible on the Earth's surface (see Li et al., 2024). Its δ18Ocarb and δ13Ccarb values fall within the range of δ18Ocarb and δ13Ccarb values observed in the other micritic nodules of this section. However, as a note of caution, we rejected this sample from further interpretation, even though the inclusion of the sample in our analysis would not change our interpretation and conclusions.
4.2 Paleosol temperature reconstruction
We report stable and clumped isotope data of our DVB record within four intervals, which closely follow Miocene stages (Fig. 3). Interval I (Aquitanian, 22.3–21.1 Ma) yields relatively similar T(Δ47) values with an average temperature of 27.6 °C (n=2; SD=1.6 °C), while data from Interval II (Burdigalian, 19.6–16.7 Ma) show positive trends in δ13Ccarb (from ca. −7.4 ‰ to −6.9 ‰) and δ18Ocarb values (from ca. −6.6 ‰ to −6.0 ‰) and an average Δ47 temperature of 32.0 °C (n=4; SD=3.5 °C; Fig. S3). Interval III (Langhian–Early Serravallian, 16.0–13.8 Ma) is characterized by high-amplitude and rapid temperature variations ranging from 20.6–34.7 °C (n=7; SD=5.3 °C; Fig. 3), with corresponding changes in the δ18Ow record. Overall, δ13Ccarb and δ18Ocarb values follow a positive trend from 18.6–14.5 Ma (Fig. S3).
Interval IV (Serravallian, 13.4–13.2 Ma) is characterized by the two lowest Δ47 temperatures in our record of 20.5 and 16.8 °C (13.4 and 13.2 Ma, respectively; average 18.6 °C; n=2; SD=2.6 °C).
In conclusion, we observe the following patterns in our Miocene record. The Aquitanian data reflect similar temperatures averaging 27.6 °C. During the Burdigalian and the Langhian, we observe three key elements in our data: (a) Δ47 temperatures decrease from ca. 33 °C (18.3 Ma) to 20 °C (15.0 Ma), (b) Δ47 temperatures increase to ca. 34 °C at the end of the Langhian and the start of the Serravallian, and (c) Δ47 temperatures decrease at 13.8 Ma to the lowest measured Δ47 temperatures. The rapid large magnitude decrease in Δ47 temperature from 34.3–16.8 °C between 13.8 and 13.2 Ma is accompanied by a 1.5 ‰ decrease in δ18Ocarb values from −5.5 ‰ to −7.0 ‰, which corresponds to a decrease in δ18Ow values of 5.1 ‰ from −1.0 ‰ to −6.1 ‰.
5.1 Pristine stable isotope values
We consider the obtained Δ47 temperatures pristine and to reflect pedogenic carbonate growth temperatures for the following reasons: (1) our Δ47 temperatures align with the range of temperatures found in the upper layers of Earth's subsurface, which are mainly controlled by the MAT (Molnar, 2022; Mosbrugger et al., 2005); (2) within the reported samples there is no evidence of (localized) fluid flow and associated recrystallization (Quade et al., 2013, and references therein), which is attested by thin sections of nodules that reveal a dominantly micritic texture (Fig. S1); (3) the δ18Ocarb values fall into the range attributed to unaltered pedogenic carbonates (Tabor and Myers, 2015); and (4) the burial depth of the sampled paleosol sequences in the DVB was relatively shallow (<500 m; Ford et al., 1999), and there is no overall trend to higher temperatures within the more deeply buried parts of the section.
However, one pedogenic carbonate sample (99GR22) contains sparry calcite veins. This sample may have been affected by diagenetic alteration, and its relatively high Δ47 temperature (39.2 °C; Table S1; Fig. S2) may not reflect pedogenic carbonate formation temperatures but rather carbonate formation during diagenesis. Consequently, the sample was not included in the Discussion.
5.2 Seasonality of soil carbonate precipitation
We observe large (up to 17.5 °C) variations in T(Δ47) soil carbonate formation temperatures in the DVB record. Owing to the fact that the sampling depth of carbonate nodules does not exceed 1 m below the preserved surface of the paleosol, we rule out the possibility that changes in depth associated with fluctuations in precipitation amount significantly affect the measured T(Δ47) (Kelson et al., 2020; Molnar, 2022). Such large temperature variations cannot be explained by regional or global terrestrial temperature change alone (e.g., Fauquette et al., 2015; Mosbrugger et al., 2005; Westerhold et al., 2020).
In situ pedogenic carbonate formation occurs within soil profiles in the presence of soil water in semi-arid to sub-humid climates. Pedogenic carbonates most commonly form during the warm and dry season when plants are active and evapotranspiration attains a maximum (Quade et al., 2007, 2013; Zamanian et al., 2016). As such, carbonate precipitation responds to interannual changes in soil moisture availability and is mainly controlled by the interplay between soil water availability, soil temperature, and soil CO2 levels in the presence of sufficient Ca2+. Net calcite accumulation occurs when (re-)precipitation of calcite exceeds calcite dissolution during seasonal wet and dry cycling (Zamanian et al., 2016). This implies that changes in the timing and/or duration of the pedogenic carbonate growth season will affect Δ47 temperatures. Because soil drying is an important requirement for pedogenic carbonate formation, a change in the timing of the dry season would inevitably result in a shift in the pedogenic carbonate formation period (Fig. 4). Consequently, recorded Δ47 temperatures may vary depending on the timing of rainfall seasonality and hence seasonality of carbonate formation (Fig. 4a and b; Gallagher et al., 2019; Kelson et al., 2020). In accordance with other studies of pedogenic carbonate T(Δ47) from central Europe (e.g., Methner et al., 2020), we suggest that a seasonal change in the timing of annual carbonate precipitation may have played an important role in controlling the observed temperature pattern, i.e., a change in pedogenic carbonate precipitation from the cool(er) to the warm(er) season or vice versa. Presently, summer temperatures average ca. 19 °C and winter temperatures average ca. 2 °C in the DVB (IAEA/WMO, 2024). Soil temperatures are typically higher than air temperatures due to solar radiation heating (Molnar, 2022; Quade et al., 2013). At shallow depths (<30 cm), soil temperature exhibits significant daily fluctuations, but these diurnal variations diminish with depth. Beyond 300 cm, soil temperature becomes nearly constant throughout the year, converging toward the MAT (Molnar, 2022; Quade et al., 2013; Sheldon and Tabor, 2009). A hypothetical instantaneous shift from warm- to cold-season carbonate precipitation or vice versa would therefore presently account for a change of up to 17 °C in soil carbonate formation temperatures.
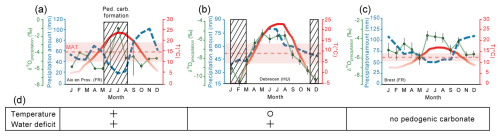
Figure 4Present-day climate conditions for three locations in Europe: (a, c) Aix en Provence and Brest (France) and (b) Debrecen (Hungary). Variations in seasonality of precipitation may drive changes in pedogenic carbonate formation temperatures. Depending on the seasonality of pedogenic carbonate formation, soil temperatures reconstructed from pedogenic carbonate should reflect (a) warm (>MAT) or (b) cool (≤MAT) season temperatures. In panel (c), conditions for the formation of pedogenic carbonate are not met because of the lack of contrasting rainfall seasonality and temperature throughout the year; hence no pedogenic carbonate is formed at this location. The δ18Ow values are retrieved from the GNIP stations near the locations. (d) Influence (cross: large; circle: moderate) of the interplay between soil temperature and soil water deficit on the formation of pedogenic carbonate. Temperature-dominated (a) and water-deficit-dominated (b) scenarios and a scenario with no pedogenic carbonate formation (c).
Based on the above, we suggest that changes in the Δ47 soil carbonate formation temperatures throughout the Middle Miocene are best explained by warming or cooling during the MCO and MMCT, respectively, in combination with modifications of the intra-annual timing of soil carbonate formation.
A change in seasonality of carbonate precipitation explaining the large observed temperature variations in our Δ47 record is supported by the large variations in calculated δ18Ow during the MCO and MMCT of up to 3.8 ‰ and 5.0 ‰, respectively. These δ18Ow variations may reflect changes in atmospheric circulation patterns and/or a change in the season of moisture delivery, which would both be associated with different moisture sources. Shifts in the seasonality of pedogenic carbonate formation could potentially be associated with variations in δ13Ccarb values. We suggest that δ13C of pedogenic carbonates would decrease with rising temperatures, primarily due to increased biological activity and CO2 production. However, isotopic fractionation during carbonate precipitation moderates this relationship, making it a complex interaction between biological and geochemical processes. However, we would expect lower δ13Ccarb values during time periods associated with cooler temperatures and high precipitation amounts. This agrees with the significantly low δ13Ccarb values during the MMCT in our record (Fig. 3). Consequently, a change in the seasonal timing of precipitation in the DVB is likely to be linked with a change in moisture source(s) over the region.
A shift in precipitation seasonality over western (this study) and central Europe (Methner et al., 2020) throughout the Middle Miocene requires a profound reorganization of atmospheric circulation. The distribution of fossil snakehead fish at the start of the MCO suggests moist summer conditions which may be reached by a poleward shift in the Hadley circulation (Böhme, 2004). This may have resulted in a southeastward transport of air masses over the North Sea basin and the Paratethys and along the Alpine chain transporting moist air over central Europe during the summer months (Böhme, 2004), which is supported by tree fall directions and the eccentricity of tree growth rings (Utescher and Bruch, 2000). After the MCO, a permanent shift from a northeasterly to a westerly dominated wind regime (similar to the present-day Mediterranean-type climate) over the northern Mediterranean region is suggested to have occurred at the latest before the Tortonian (ca. 12 Ma; Quan et al., 2014).
The data presented here do not permit the identification of drivers and mechanisms of changes in atmospheric circulation, but we consider it likely that an alternation between northeasterly winds and westerlies or latitudinal variability in the main westerlies track throughout the MCO contributed to modifying the pattern of wet and dry seasons and hence the temperature fluctuations observed in our Δ47 soil carbonate data.
We suggest that the DVB MCO and MMCT record reveals two distinctly different climatic patterns. During the Langhian, the climate is marked by a dry, cool season. Soil-drying and pedogenic carbonate formation during late fall and/or early winter (Fig. 4b) are reflected in relatively low T(Δ47) and δ18Ow values. In this “dry winter” scenario, rainfall occurred mostly during warmer (summer) months and was possibly delivered by moist air masses from the North Atlantic Ocean. We hypothesize that a transition to a climate similar to the present-day Mediterranean-type climate (“proto-Mediterranean climate”), characterized by hot and dry summers (Fig. 4a), occurred during the Middle Miocene (at ca. 16.5 and 14.2–13.8 Ma; Fig. 5). This implies that, already during certain time intervals of the MCO, a climate similar to the present-day Mediterranean climate existed. In such a climate state, the drying of soils during the summer season led to dominant pedogenic carbonate formation in early summer, with associated high T(Δ47) and δ18Ow values. Finally, our data are consistent with a return to the “dry winter” scenario in the early Serravallian (13.4–13.2 Ma), resulting in relatively low Δ47 temperatures and δ18Ow values. We therefore propose that high T(Δ47) (>30 °C) and high δ18Ow values () during the MCO and MMCT resulted from a Mediterranean moisture source (“proto-Mediterranean climate”), whereas lower Δ47 temperatures and lower δ18Ow values () reflect predominance of a North Atlantic moisture source.
5.3 Middle Miocene northern Mediterranean and central European terrestrial paleoclimate
Irrespective of the change in the seasonality of the soil carbonate formation, three main elements characterize our paleosol temperature data and include (1) the transition into the MCO (17.3–16.7 Ma) during the Burdigalian, which is reflected by an increase in Δ47 temperatures and the highest temperature of the record (35.2 °C); (2) overall cooling from the onset to the end of the MCO; (3) a large temperature increase during the MMCT; and (4) a distinct (>15 °C) and rapid (ca. 600 ka) cooling phase accompanied by a significant decrease in δ18Ow (−5.0 ‰) after ca. 13.8 Ma, which coincides with global post-MMCT cooling.
In the following, we compare the DVB record with time-equivalent terrestrial data from the northern Mediterranean region and central Europe (Fig. 5).
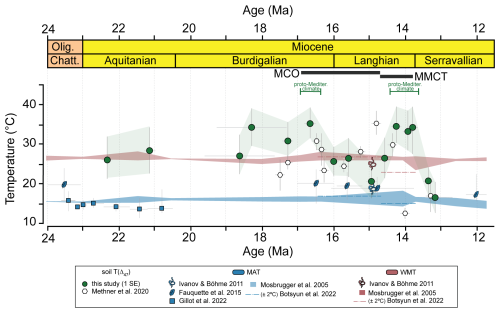
Figure 5Reconstructed Miocene mid-latitude terrestrial temperatures from western and central Europe. Temperature reconstructions based on carbonate clumped isotope thermometry from the Digne–Valensole basin are compared to T(Δ47) data from the NAFB (Methner et al., 2020), mid-latitude European mean annual temperatures (MATs) and warm-month temperatures (WMTs) inferred from terrestrial paleofloral data (Fauquette et al., 2015; Mosbrugger et al., 2005) and herpetofauna and climofunction data from paleosols (Ivanov and Böhme, 2011; Gillot et al., 2022), and modeled MAT and WMT values (Botsyun et al., 2022) for the paleogeographic position of the DVB. Timing for the MCO and MMCT is indicated, as are the two intervals of proto-Mediterranean climate in the DVB region.
During the Aquitanian (22.3–21.1 Ma) and Middle Burdigalian (18.6–17.3 Ma), T(Δ47) values of the DVB region are significantly (up to 10 °C) higher than estimated mean annual temperatures (MATs) for the DVB derived from pollen (Fauquette et al., 2015) and paleosol chemistry (Gillot et al., 2022). During the same time interval, our T(Δ47) values are also up to 10 °C higher than MAT reconstructed from central European megafloras (fruits, seeds, and leaves) but in good agreement with estimated warm-month temperatures (WMTs) (Mosbrugger et al., 2005; Fig. 5).
At the onset of the MCO, our DVB T(Δ47) record shows a warming trend similar to that observed in the T(Δ47) record from the Swiss NAFB paleosols (Methner et al., 2020).
At ca. 15 Ma, we observe colder Δ47 temperatures that align particularly well with MAT values derived from herpetological data (Ivanov and Böhme, 2011), pollen records (Fauquette et al., 2015), and climate models (Botsyun et al., 2022). This T(Δ47) value in the DVB suggests a switch from a dry-season summer bias towards conditions indicating a “dry winter” scenario with late fall/early winter soil carbonate formation (see Sect. 5.2). Conversely, during the subsequent warming trend leading into the onset of the MMCT (ca. 15.5–14.0 Ma), we observe T(Δ47) values that converge towards temperatures above the paleobotanical WMT values and hence a return to dry summers and warm-season-biased temperatures .
Finally, we attribute the large-magnitude temperature decrease after ca. 14 Ma to post-MMCT continental cooling. The climate change after 14 Ma is expressed in an impoverishment in plant diversity in western Europe (Jiménez-Moreno and Suc, 2007) and led to the regional extinction of temperature-sensitive tetrapods in central Europe, such as alligators, giant turtles, and chameleons (Ivanov and Böhme, 2011). The temperatures in the DVB at 13.5 Ma, following the post-MMCT cooling, are equivalent to the NAFB Δ47 soil temperatures and herpetological, botanical, and model-based MAT (Fig. 5; Botsyun et al., 2022; Fauquette et al., 2015; Ivanov and Böhme, 2011; Methner et al., 2020). We can only attempt to decipher the contributions of seasonal temperature change and overall regional cooling to the ca. 17.5 °C decrease in soil carbonate formation temperatures visible in the DVB Δ47 record. Assuming that the temperature change of ca. 14 °C (20.6–34.7 °C) that is observed between 14.9 and 14.2 Ma reflects only the change in carbonate formation season without any major regional temperature change, we posit any fluctuations in carbonate formation temperatures larger than 14±2 °C must be the result of additional global warming or cooling. We therefore propose that the remaining 3–4 °C temperature decrease after 13.8 Ma reflects the effect of regional post-MMCT cooling. This value is comparable to the drop in the minimal summer temperature of>7 °C between 14.0 and 13.5 Ma described in herpetological data for central Europe (Böhme, 2003). While the analytical error (1 SE) for the clumped isotope temperatures is on the order of a few degrees, the concurrent major decreases in δ13Ccarb, δ18Ocarb, and δ18Ow values within a relatively short timescale (<600 ka) suggest considerable climatic change. Therefore, the significant decrease (17.5 °C) in Δ47 temperatures and associated decrease in δ13Ccarb, δ18Ocarb, and calculated δ18Ow values at or after 13.9 Ma support the interpretation that regional cooling (3–4 °C) superimposed on a component of changing climate seasonality is accountable for the decrease in T(Δ47) (Methner et al., 2020).
5.4 Continental soil temperatures related to marine records
Global climate change would not only lead to a decrease (or increase) in marine and continental temperatures, but may also significantly impact atmospheric circulation, resulting in altered weather patterns and ocean–atmosphere interactions (Holbourn et al., 2005, 2007; Methner et al., 2020) similar to changes observed during anthropogenic warming. Changes in temperature gradients can weaken or shift trade winds, disrupting weather systems and ocean currents (Comas-Bru et al., 2016). Throughout the MCO and MMCT, our northern Mediterranean terrestrial soil Δ47 temperatures show significant similarities with marine temperature records (Fig. 6). Because the rapid and large changes in measured soil carbonate T(Δ47) during the Middle Miocene are too large to be explained by regional/global cooling (or warming) alone, we suggest that the changes in soil Δ47 temperatures result from the combined effect of changes in seasonality of rainfall and regional/global cooling (or warming).
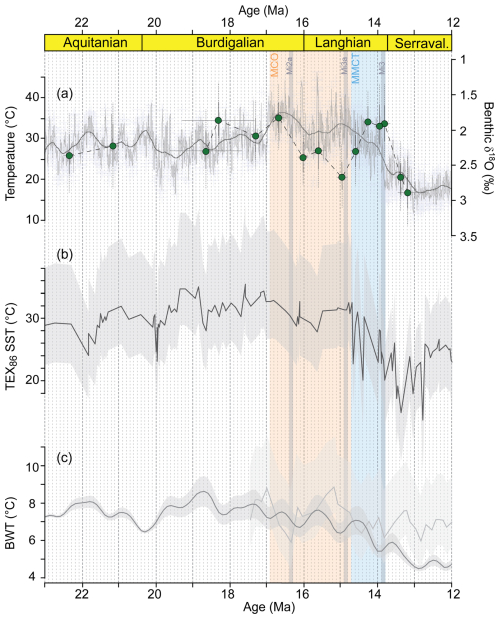
Figure 6Clumped-isotope-based reconstruction of terrestrial temperatures compared with global marine data, within the timing of the MCO, MMCT (Westerhold et al., 2020), and Mi events (Miller et al., 2020). (a) Δ47 temperatures from the Digne–Valensole basin (in green) and benthic oxygen isotopic curve (Westerhold et al., 2020). (b) TEX86-based sea surface temperatures of ODP Site 608, North Atlantic (Super et al., 2018). (c) Bottom-water temperatures from Cramer et al. (2011) in dark gray and Lear et al. (2015) in light gray.
The MCO is characterized as a period of sustained global warmth marked by high-amplitude climate variability (Fig. 6a) (Holbourn et al., 2007, 2014, 2015; Steinthorsdottir et al., 2021b). Our terrestrial record agrees with global records and indicates overall high temperatures with pronounced temperature variability throughout the MCO.
Following a period of rapid warming and/or polar ice melting at 16.9 Ma at the onset of the MCO, the Middle Miocene was globally marked by fundamental changes in the global carbon reservoir, resulting in δ18Obenthic and δ13Cbenthic increases (Fig. 6a) (Holbourn et al., 2015, 2022; Westerhold et al., 2020) and cooling in sea surface (Fig. 6b) and bottom-water temperature (BWT; Fig. 6c) from 16.5 to ca. 16.0 Ma (Cramer et al., 2011; Lear et al., 2015; Super et al., 2018). Those results align well with the cooling phase recorded in our T(Δ47) between circa 16.7 and 15.6 Ma.
Within age uncertainty, our coolest Δ47 temperature within the MCO coincides with δ18Obenthic excursion Mi3a, which is associated with significant changes in global temperature and ice-volume history (Fig. 6a and c; Holbourn et al., 2007; Miller et al., 2020).
The subsequent T(Δ47) warming trend in the MMCT from 14.9 until 14.2 Ma in our continental record is also observed in benthic records (Fig. 6a and c; Cramer et al., 2011; Westerhold et al., 2020). Finally, our rapid post-MMCT cooling (after ca. 13.8 Ma) is associated with a major decrease in δ13Ccarb (−4.0 %), δ18Ocarb (−2.5 %), and δ18Ow (−5.0 %) values and parallels the stabilization of the East Antarctic Ice Sheet, which is expressed in benthic δ18O excursion Mi3, associated sea level decline, and a decrease in BWT (Holbourn et al., 2015; Miller et al., 2020; Sosdian et al., 2020; Steinthorsdottir et al., 2021b; Westerhold et al., 2020). It also coincides particularly well in time with cooling in continuous marine successions from the eastern Mediterranean region at 13.82±0.03 Ma (Abels et al., 2005).
Results of our study documenting the paleosol Δ47 temperatures of the Digne–Valensole basin suggest the region was affected by the Middle Miocene climate dynamics similarly to the global oceans, in addition to significant and rapid changes in temperature and seasonality as a result of a reorganization in atmospheric circulation.
Our Early to Middle Miocene terrestrial T(Δ47), δ13Ccarb, δ18Ocarb, and δ18Ow record for the northern Mediterranean Digne–Valensole basin (SE France) shows the following:
-
An abrupt decrease in Δ47 temperature (17.5 °C within 600 ka) occurs at 13.8 Ma, which we attribute to post-MMCT cooling whose timing is in agreement with global marine (Holbourn et al., 2022; Westerhold et al., 2020) and Mediterranean (Abels et al., 2005) records. The decrease in Δ47 temperatures associated with a major decrease in δ13Ccarb (−4.0 %), δ18Ocarb (−2.5 %), and δ18Ow (−5.0 %) values within less than 600 ka may be best explained by a large seasonality component and overall cooling on the order of 3–4 °C.
-
During the MCO, DVB Δ47 temperatures are higher than other reconstructed European mid-latitudinal average warm-month temperatures (Ivanov and Böhme, 2011; Mosbrugger et al., 2005).
-
Similarly to the observations from the Swiss NAFB record (Methner et al., 2020), we suggest that variations in Δ47 temperatures record the combined effect of global warming and cooling and changes in the annual distribution of rainfall, which affects the timing of pedogenic carbonate formation. The latter would result from the reorganization of atmospheric circulation in the circum-Alpine region.
-
We suggest that a proto-Mediterranean climate characterized by hot and dry summers already existed during certain time intervals of the MCO.
-
We identify an increase in soil Δ47 temperatures at 15 Ma, which coincides with warming trends observed in global marine data (Cramer et al., 2011; Westerhold et al., 2020).
-
We observe a cooling phase in our Δ47 temperatures between circa 16.7 and 15.6 Ma, which coincides with cooling in global sea surface and bottom-water temperatures (Cramer et al., 2011; Lear et al., 2015; Super et al., 2018).
-
The coolest recorded temperature during the MCO in our record coincides with the δ18Obenthic excursion Mi3a (14.8 Ma) and associated transient glacial expansion (Miller et al., 2020).
All supporting datasets are available in the Supplement.
The supplement related to this article is available online at https://doi.org/10.5194/cp-21-841-2025-supplement.
AB: writing (original draft). AB, MJMM, AM, IC, MB, DH, KM, JF, and MT: writing (review and editing). AB, MJMM, KM, and AM: conceptualization. AB and IC: investigation. AB: visualization. MJMM and AM: funding acquisition. MJMM, AM, and IC: supervision. MB: software. MB and MT: formal analysis. JF: methodology and resources.
The contact author has declared that none of the authors has any competing interests.
Publisher's note: Copernicus Publications remains neutral with regard to jurisdictional claims made in the text, published maps, institutional affiliations, or any other geographical representation in this paper. While Copernicus Publications makes every effort to include appropriate place names, the final responsibility lies with the authors.
Armelle Ballian and Maud J. M. Meijers acknowledge funding through grants from the German Science Foundation (DFG) in the form of Special Priority Program 2020 “Mountain-Building in Four-Dimensions” (DFG-SPP 4D MB). We thank Sven Hofmann for assistance with clumped isotope analyses and Alexis Licht for field assistance.
This research has been supported by the Deutsche Forschungsgemeinschaft (grant-nos. MU2845/7-1 and ME5579/1-1).
This paper was edited by Shiling Yang and reviewed by two anonymous referees.
Abels, H. A., Hilgen, F. J., Krijgsman, W., Kruk, R. W., Raffi, I., Turco, E., and Zachariasse, W. J.: Long-period orbital control on middle Miocene global cooling: Integrated stratigraphy and astronomical tuning of the Blue Clay Formation on Malta, Paleoceanography, 20, PA4012, https://doi.org/10.1029/2004PA001129, 2005.
Abels, H. A., Abdul Aziz, H., Calvo, J. P., and Tuenter, E.: Shallow lacustrine carbonate microfacies document orbitally paced lake-level history in the Miocene Teruel Basin (North-East Spain), Sedimentology, 56, 399–419, https://doi.org/10.1111/j.1365-3091.2008.00976.x, 2009.
Anderson, N. T., Kelson, J. R., Kele, S., Daëron, M., Bonifacie, M., Horita, J., Mackey, T. J., John, C. M., Kluge, T., Petschnig, P., Jost, A. B., Huntington, K. W., Bernasconi, S. M., and Bergmann, K. D.: A Unified Clumped Isotope Thermometer Calibration (0.5–1100 °C) Using Carbonate-Based Standardization, Geophys. Res. Lett., 48, 1–11, https://doi.org/10.1029/2020GL092069, 2021.
Bauer, H.: Influence du climat, de l'eustatisme et de la tectonique dans l'architecture des séries continentales: cas du Miocène inférieur et moyen du bassin de Digne-Valensole (se France), Doctoral dissertation, Paris, ENMP, https://pastel.hal.science/pastel-00002113/file/BAUER-manuscrit.pdf (last access: July 2024), 2006.
Berggren, W. A., Kent, D. V., Swisher, C. C., and Aubry, M. P.: A revised Cenozoic geochronology and chronostratigraphy, Soc. Sediment. Geol., 54, 129–212, https://doi.org/10.2110/pec.95.04.0129, 1995.
Bernasconi, S. M., Müller, I. A., Bergmann, K. D., Breitenbach, S. F. M., Fernandez, A., Hodell, D. A., Jaggi, M., Meckler, A. N., Millan, I., and Ziegler, M.: Reducing Uncertainties in Carbonate Clumped Isotope Analysis Through Consistent Carbonate-Based Standardization, Geochem. Geophy. Geosy., 19, 2895–2914, https://doi.org/10.1029/2017GC007385, 2018.
Bernasconi, S. M., Daëron, M., Bergmann, K. D., Bonifacie, M., Meckler, A. N., Affek, H. P., Anderson, N., Bajnai, D., Barkan, E., Beverly, E., Blamart, D., Burgener, L., Calmels, D., Chaduteau, C., Clog, M., Davidheiser-Kroll, B., Davies, A., Dux, F., Eiler, J., Elliott, B., Fetrow, A. C., Fiebig, J., Goldberg, S., Hermoso, M., Huntington, K. W., Hyland, E., Ingalls, M., Jaggi, M., John, C. M., Jost, A. B., Katz, S., Kelson, J., Kluge, T., Kocken, I. J., Laskar, A., Leutert, T. J., Liang, D., Lucarelli, J., Mackey, T. J., Mangenot, X., Meinicke, N., Modestou, S. E., Müller, I. A., Murray, S., Neary, A., Packard, N., Passey, B. H., Pelletier, E., Petersen, S., Piasecki, A., Schauer, A., Snell, K. E., Swart, P. K., Tripati, A., Upadhyay, D., Vennemann, T., Winkelstern, I., Yarian, D., Yoshida, N., Zhang, N., and Ziegler, M.: InterCarb: A Community Effort to Improve Interlaboratory Standardization of the Carbonate Clumped Isotope Thermometer Using Carbonate Standards, Geochem. Geophy. Geosy., 22, 1–25, https://doi.org/10.1029/2020GC009588, 2021.
Bernecker, M., Hofmann, S., Staudigel, P. T., Davies, A. J., Tagliavento, M., Meijer, N., Ballian, A., and Fiebig, J.: A robust methodology for triple (Δ47, Δ48, Δ49) clumped isotope analysis of carbonates, Chem. Geol., 642, 121803, https://doi.org/10.1016/j.chemgeo.2023.121803, 2023.
Bialkowski, A.: Stratigraphie isotopique (carbone et oxygène) des séries continentales d'un bassin d'avant-pays (Oligo-Miocène du bassin de Digne-Valensole): paléoenvironnements et séquences de dépot, École Nationale Supérieure des Mines de Paris, https://theses.hal.science/tel-00819770v1/document (last access: July 2024), 2002.
Bialkowski, A., Châteauneuf, J. J., Cojan, I., and Bauer, H.: Integrated stratigraphy and paleoenvironmental reconstruction of the Miocene series of the Châteauredon Dome, S. E. France, Eclogae Geol. Helv., 99, 1–15, https://doi.org/10.1007/s00015-006-1176-y, 2006.
Böhme, M.: The Miocene Climatic Optimum: Evidence from ectothermic vertebrates of Central Europe, Palaeogeogr. Palaeocl., 195, 389–401, https://doi.org/10.1016/S0031-0182(03)00367-5, 2003.
Böhme, M.: Migration history of air-breathing fishes reveals Neogene atmospheric circulation patterns, Geology, 32, 393–396, https://doi.org/10.1130/G20316.1, 2004.
Botsyun, S., Ehlers, T. A., Koptev, A., Böhme, M., Methner, K., Risi, C., Stepanek, C., Mutz, S. G., Werner, M., Boateng, D., and Mulch, A.: Middle Miocene Climate and Stable Oxygen Isotopes in Europe Based on Numerical Modeling, Paleoceanogr. Paleoclimatology, 37, 1–30, https://doi.org/10.1029/2022pa004442, 2022.
Châteauneuf, J. J., Bauer, H., and Cojan, I.: Présence d'une mangrove à Avicennia au Miocène moyen dans la région de Digne (Alpes-de-Haute-Provence, France): Implications stratigraphiques et paléoclimatiques, C. R. Geosci., 338, 197–205, https://doi.org/10.1016/j.crte.2005.10.004, 2006.
Cojan, I. and Gillot, T.: Paleosols in distal alluvial sequences and their formation mechanisms: Insights from a high-resolution record in a foreland basin during early Miocene (SE France), Palaeogeogr. Palaeocl., 595, 110983, https://doi.org/10.1016/j.palaeo.2022.110983, 2022.
Cojan, I., Bialkowski, A., Gillot, T., and Renard, M.: Paleoenvironnement and paleoclimate reconstruction for the early to middle Miocene from stable isotopes in pedogenic carbonates (Digne-Valensole basin, southeastern France), B. Soc. Geol. Fr., 184, 583–599, https://doi.org/10.2113/gssgfbull.184.6.583, 2013.
Comas-Bru, L., McDermott, F., and Werner, M.: The effect of the East Atlantic pattern on the precipitation δ18O-NAO relationship in Europe, Clim. Dynam., 47, 2059–2069, https://doi.org/10.1007/s00382-015-2950-1, 2016.
Costeur, L. and Legendre, S.: Mammalian communities document a latitudinal environmental gradient during the Miocene Climatic Optimum in western Europe, Palaios, 23, 280–288, https://doi.org/10.2110/palo.2006.p06-092r, 2008.
Cramer, B. S., Miller, K. G., Barrett, P. J., and Wright, J. D.: Late Cretaceous-Neogene trends in deep ocean temperature and continental ice volume: Reconciling records of benthic foraminiferal geochemistry (δ18O and MgCa) with sea level history, J. Geophys. Res.-Oceans, 116, 1–23, https://doi.org/10.1029/2011JC007255, 2011.
Daëron, M.: Full Propagation of Analytical Uncertainties in Δ47 Measurements, Geochem. Geophy. Geosy., 22, 1–19, https://doi.org/10.1029/2020GC009592, 2021.
Eiler, J. M.: Paleoclimate reconstruction using carbonate clumped isotope thermometry, Quaternary Sci. Rev., 30, 3575–3588, https://doi.org/10.1016/j.quascirev.2011.09.001, 2011.
Fauquette, S., Suc, J.-P., Jiménez-Moreno, G., Micheels, A., Jost, A., Favre, E., Bachiri-Taoufiq, N., Bertini, A., Clet-Pellerin, M., Diniz, F., Farjanel, G., Feddi, N., and Zheng, Z.: Latitudinal climatic gradients in the Western European and Mediterranean regions from the Mid-Miocene (c. 15 Ma) to the Mid-Pliocene (c. 3.5 Ma) as quantified from pollen data, Deep-Time Perspectives on Climate Change: Marrying the Signal from Computer Models and Biological Proxies, edited by: Williams, M., Haywood, A. M., Gregory, F. J., and Schmidt, D. N., Geological Society of London, https://doi.org/10.1144/tms002.22, 2007.
Fauquette, S., Bernet, M., Suc, J. P., Grosjean, A. S., Guillot, S., van der Beek, P., Jourdan, S., Popescu, S. M., Jiménez-Moreno, G., Bertini, A., Pittet, B., Tricart, P., Dumont, T., Schwartz, S., Zheng, Z., Roche, E., Pavia, G., and Gardien, V.: Quantifying the Eocene to Pleistocene topographic evolution of the southwestern Alps, France and Italy, Earth Planet. Sc. Lett., 412, 220–234, https://doi.org/10.1016/j.epsl.2014.12.036, 2015.
Fiebig, J., Daëron, M., Bernecker, M., Guo, W., Schneider, G., Boch, R., Bernasconi, S. M., Jautzy, J., and Dietzel, M.: Calibration of the dual clumped isotope thermometer for carbonates, Geochim. Cosmochim. Ac., 312, 235–256, https://doi.org/10.1016/j.gca.2021.07.012, 2021.
Flower, B. P. and Kennett, J. P.: The middle Miocene climatic transition: East Antarctic ice sheet development, deep ocean circulation and global carbon cycling, Palaeogeogr. Palaeocl., 108, 537–555, https://doi.org/10.1016/0031-0182(94)90251-8, 1994.
Ford, M., Lickorish, W. H., and Kusznir, N. J.: Tertiary foreland sedimentation in the Southern Subalpine Chains, SE France: A geodynamic appraisal, Basin Res., 11, 315–336, https://doi.org/10.1046/j.1365-2117.1999.00103.x, 1999.
Gallagher, T. M., Hren, M., and Sheldon, N. D.: The effect of soil temperature seasonality on climate reconstructions from paleosols, Am. J. Sci., 319, 549–581, https://doi.org/10.2475/07.2019.02, 2019.
Ghosh, P., Adkins, J., Affek, H., Balta, B., Guo, W., Schauble, E. A., Schrag, D., and Eiler, J. M.: 13C-18O bonds in carbonate minerals: A new kind of paleothermometer, Geochim. Cosmochim. Acta, 70, 1439–1456, https://doi.org/10.1016/j.gca.2005.11.014, 2006.
Gigot, P., Grandjacquet, C., and Haccard, D.: Évolution tectono-sédimentaire de la bordure septentrionale du bassin tertiaire de Digne depuis l'Éocène, B. Soc. Geol. Fr., XVI, 128–139, 1974.
Gile, L. H., Peterson, F. F., and Grossman, R. B.: Morphological and genetic sequences of carbonate accumulation in desert soils, Soil Sci., 101, 347–360, https://doi.org/10.4324/9781315844855, 1966.
Gillot, T., Cojan, I., and Badía, D.: Paleoclimate instabilities during late Oligocene – Early Miocene in SW Europe from new geochemical climofunctions based on soils with pedogenic carbonate, Palaeogeogr. Palaeocl., 591, 110882, https://doi.org/10.1016/j.palaeo.2022.110882, 2022.
IAEA/WMO: Global Network of Isotopes in Precipitation. The GNIP Database, https://nucleus.iaea.org/wiser (last access: December 2024), 2024.
Holbourn, A., Kuhnt, W., Schulz, M., and Erlenkeuser, H.: Impacts of orbital forcing and atmospheric carbon dioxide on Miocene ice-sheet expansion, Nature, 438, 483–487, https://doi.org/10.1038/nature04123, 2005.
Holbourn, A., Kuhnt, W., Schulz, M., Flores, J. A., and Andersen, N.: Orbitally-paced climate evolution during the middle Miocene “Monterey” carbon-isotope excursion, Earth Planet. Sc. Lett., 261, 534–550, https://doi.org/10.1016/j.epsl.2007.07.026, 2007.
Holbourn, A., Kuhnt, W., Lyle, M., Schneider, L., Romero, O., and Andersen, N.: Middle Miocene climate cooling linked to intensification of eastern equatorial Pacific upwelling, Geology, 42, 19–22, https://doi.org/10.1130/G34890.1, 2014.
Holbourn, A., Kuhnt, W., Kochhann, K. G. D., Andersen, N., and Meier, K. J. S.: Global perturbation of the carbon cycle at the onset of the Miocene Climatic Optimum, Geology, 43, 123–126, https://doi.org/10.1130/G36317.1, 2015.
Holbourn, A. E., Kuhnt, W., Kochhann, K. G. D., Matsuzaki, K. M., and Andersen, N.: Middle Miocene climate-carbon cycle dynamics: keys for understanding future trends on a warmer Earth? In Undertanding the Monterey Formation and Similar Biosiliceous Units Across Space and Time, Geol. Soc. Am. Sp. P., 556, 1–19, https://doi.org/10.1130/2022.2556(05), 2022.
Ivanov, M. and Böhme, M.: Snakes from Griesbeckerzell (Langhian, Early Badenian), North Alpine Foreland Basin (Germany), with comments on the evolution of snake faunas in Central Europe during the Miocene Climatic Optimum, Geodiversitas, 33, 411–449, https://doi.org/10.5252/g2011n3a2, 2011.
Jiménez-Moreno, G. and Suc, J.-P.: Middle Miocene latitudinal climatic gradient in Western Europe: Evidence from pollen records, Palaeogeogr. Palaeocl., 253, 208–225, https://doi.org/10.1016/j.palaeo.2007.03.040, 2007.
Jiménez-Moreno, G., Rodríguez-Tovar, F. J., Pardo-Igúzquiza, E., Fauquette, S., Suc, J. P., and Müller, P.: High-resolution palynological analysis in late early-middle Miocene core from the Pannonian Basin, Hungary: Climatic changes, astronomical forcing and eustatic fluctuations in the Central Paratethys, Palaeogeogr. Palaeocl., 216, 73–97, https://doi.org/10.1016/j.palaeo.2004.10.007, 2005.
Jiménez-Moreno, G., Fauquette, S., and Suc, J.-P.: Vegetation, climate and palaeoaltitude reconstructions of the Eastern Alps during the Miocene based on pollen records from Austria, Central Europe, J. Biogeogr., 35, 1638–1649, https://doi.org/10.1111/j.1365-2699.2008.01911.x, 2008.
Joly, D., Brossard, T., Cardot, H., Cavailhès, J., Hilal, M., and Wavresky, P.: Les types de climats en France, une construction spatiale, Rev. Eur. géographie/Eur. J. Geogr., 501, 1–23, 2010.
Kälin, D. and Kempf, O.: High-resolution stratigraphy from the continental record of the Middle Miocene Northern Alpine Foreland Basin of Switzerland, Neues Jahrb. Geol. P.-A., 254, 177–235, https://doi.org/10.1127/0077-7749/2009/0010, 2009.
Kelson, J. R., Watford, D., Bataille, C., Huntington, K. W., Hyland, E., and Bowen, G. J.: Warm Terrestrial Subtropics During the Paleocene and Eocene: Carbonate Clumped Isotope (Δ47) Evidence From the Tornillo Basin, Texas (USA), Paleoceanogr. Paleoclimatology, 33, 1230–1249, https://doi.org/10.1029/2018PA003391, 2018.
Kelson, J. R., Huntington, K. W., Breecker, D. O., Burgener, L. K., Gallagher, T. M., Hoke, G. D., and Petersen, S. V.: A proxy for all seasons? A synthesis of clumped isotope data from Holocene soil carbonates, Quaternary Sci. Rev., 234, 106259, https://doi.org/10.1016/j.quascirev.2020.106259, 2020.
Kim, S. T. and O'Neil, J. R.: Equilibrium and nonequilibrium oxygen isotope effects in synthetic carbonates, Geochim. Cosmochim. Ac., 61, 3461–3475, https://doi.org/10.1016/S0016-7037(97)00169-5, 1997.
Kotov, S. and Paelike, H.: QAnalySeries – a cross-platform time series tuning and analysis tool, AGU Fall Meeting Abstracts, Washington DC, 10–14 December 2018, American Geophysical Union, PP53D-1230, https://ui.adsabs.harvard.edu/abs/2018AGUFMPP53D1230K/abstract (last access: July 2024), 2018.
Lear, C. H., Coxall, H. K., Foster, G. L., Lunt, D. J., Mawbey, E. M., Rosenthal, Y., Sosdian, S. M., Thomas, E., and Wilson, P. A.: Neogene ice volume and ocean temperatures: Insights from infaunal foraminiferal Mg/Ca paleothermometry, Paleoceanography, 30, 1437–1454, https://doi.org/10.1002/2015PA002833, 2015.
Li, L., Quade, J., Garzione, C., Defliese, W. F., DeCelles, P., and Kapp, P.: Reliability of micritic carbonates in recording well-preserved isotopic composition and implications for paleoelevation estimates in central Tibet, Geochim. Cosmochim. Ac., 375, 186–200, https://doi.org/10.1016/j.gca.2024.04.009, 2024.
Lionello, P.: The Climate of the Mediterranean Region: From the Past to the Future, Elsevier, 1–502, ISBN 978-0-12-416042-2, 2012.
Longman, J., Mills, B. J. W., Donnadieu, Y., and Goddéris, Y.: Assessing Volcanic Controls on Miocene Climate Change, Geophys. Res. Lett., 49, 1–11, https://doi.org/10.1029/2021GL096519, 2022.
Meijer, N., Licht, A., Woutersen, A., Hoorn, C., Robin-Champigneul, F., Rohrmann, A., Tagliavento, M., Brugger, J., Kelemen, F. D., Schauer, A. J., Hren, M. T., Sun, A., Fiebig, J., Mulch, A., and Dupont-Nivet, G.: Proto-monsoon rainfall and greening in Central Asia due to extreme early Eocene warmth, Nat. Geosci., 17, 158–164, https://doi.org/10.1038/s41561-023-01371-4, 2024.
Methner, K., Campani, M., Fiebig, J., Löffler, N., Kempf, O., and Mulch, A.: Middle Miocene long-term continental temperature change in and out of pace with marine climate records, Sci. Rep.-UK, 10, 1–10, https://doi.org/10.1038/s41598-020-64743-5, 2020.
Miller, K. G., Wright, J. D., and Fairbanks, R. G.: Unlocking the ice house: Oligocene-Miocene oxygen isotopes, eustasy, and margin erosion, J. Geophys. Res., 96, 6829–6848, https://doi.org/10.1029/90JB02015, 1991.
Miller, K. G., Browning, J. V., John Schmelz, W., Kopp, R. E., Mountain, G. S., and Wright, J. D.: Cenozoic sea-level and cryospheric evolution from deep-sea geochemical and continental margin records, Sci. Adv., 6, https://doi.org/10.1126/sciadv.aaz1346, 2020.
Molnar, P.: Differences between soil and air temperatures: Implications for geological reconstructions of past climate, Geosphere, 18, 800–824, https://doi.org/10.1130/GES02448.1, 2022.
Mosbrugger, V., Utescher, T., and Dilcher, D. L.: Cenozoic continental climatic evolution of Central Europe, P. Natl. Acad. Sci. USA, 102, 14964–14969, https://doi.org/10.1073/pnas.0505267102, 2005.
Mudelsee, M., Bickert, T., Lear, C. H., and Lohmann, G.: Cenozoic climate changes: A review based on time series analysis of marine benthic δ18O records, Rev. Geophys., 52, 333–374, https://doi.org/10.1002/2013RG000440, 2014.
Pound, M. J., Haywood, A. M., Salzmann, U., and Riding, J. B.: Global vegetation dynamics and latitudinal temperature gradients during the Mid to Late Miocene (15.97–5.33 Ma), Earth-Sci. Rev., 112, 1–22, https://doi.org/10.1016/j.earscirev.2012.02.005, 2012.
Quade, J., Garzione, C., and Eiler, J.: Paleoelevation reconstruction using pedogenic carbonates, Rev. Mineral. Geochem., 66, 53–87, https://doi.org/10.2138/rmg.2007.66.3, 2007.
Quade, J., Eiler, J., Daëron, M., and Achyuthan, H.: The clumped isotope geothermometer in soil and paleosol carbonate, Geochim. Cosmochim. Ac., 105, 92–107, https://doi.org/10.1016/j.gca.2012.11.031, 2013.
Quan, C., Liu, Y. S. C., Tang, H., and Utescher, T.: Miocene shift of European atmospheric circulation from trade wind to westerlies, Sci. Rep.-UK, 4, 1–6, https://doi.org/10.1038/srep05660, 2014.
Retallack, G. J.: Pedogenic carbonate proxies for amount and seasonality of precipitation in paleosols, Geology, 33, 333–336, https://doi.org/10.1130/G21263.1, 2005.
Rugenstein, J. K. C., Methner, K., Kukla, T., Mulch, A., Lüdecke, T., Fiebig, J., Meltzer, A., Wegmann, K. W., Zeitler, P., and Chamberlain, C. P.: Clumped Isotope Constraints on Warming and Precipitation Seasonality in Mongolia Following Altai Uplift, Am. J. Sci., 322, 28–54, https://doi.org/10.2475/01.2022.02, 2022.
Seager, R., Osborn, T. J., Kushnir, Y., Simpson, I. R., Nakamura, J., and Liu, H.: Climate variability and change of mediterranean-type climates, J. Climate, 32, 2887–2915, https://doi.org/10.1175/JCLI-D-18-0472.1, 2019.
Sheldon, N. D. and Tabor, N. J.: Quantitative paleoenvironmental and paleoclimatic reconstruction using paleosols, Earth-Sci. Rev., 95, 1–52, https://doi.org/10.1016/j.earscirev.2009.03.004, 2009.
Sosdian, S. M., Babila, T. L., Greenop, R., Foster, G. L., and Lear, C. H.: Ocean Carbon Storage across the middle Miocene: a new interpretation for the Monterey Event, Nat. Commun., 11, 1–11, https://doi.org/10.1038/s41467-019-13792-0, 2020.
Steinthorsdottir, M., Jardine, P. E., and Rember, W. C.: Near-Future pCO2 During the Hot Miocene Climatic Optimum, Paleoceanogr. Paleoclimatology, 36, e2020PA003900, https://doi.org/10.1029/2020PA003900, 2021a.
Steinthorsdottir, M., Coxall, H. K., de Boer, A. M., Huber, M., Barbolini, N., Bradshaw, C. D., Burls, N. J., Feakins, S. J., Gasson, E., Henderiks, J., Holbourn, A. E., Kiel, S., Kohn, M. J., Knorr, G., Kürschner, W. M., Lear, C. H., Liebrand, D., Lunt, D. J., Mörs, T., Pearson, P. N., Pound, M. J., Stoll, H., and Strömberg, C. A. E.: The Miocene: The Future of the Past, Paleoceanogr. Paleoclimatology, 36, e2020PA004037, https://doi.org/10.1029/2020PA004037, 2021b.
Suc, J.-P.: Origin and evolution of the Mediterranean vegetation and climate in Europe, Nature, 307, 429–432, 1984.
Super, J. R., Thomas, E., Pagani, M., Huber, M., O'Brien, C., and Hull, P. M.: North Atlantic temperature and pCO2 coupling in the early-middle Miocene, Geology, 46, 519–522, https://doi.org/10.1130/G40228.1, 2018.
Tabor, N. J. and Myers, T. S.: Paleosols as indicators of paleoenvironment and paleoclimate, Annu. Rev. Earth Pl. Sc., 43, 333–361, https://doi.org/10.1146/annurev-earth-060614-105355, 2015.
Ulbrich, U., Lionello, P., Belušić, D., Jacobeit, J., Knippertz, P., Kuglitsch, F. G., Leckebusch, G. C., Luterbacher, J., Maugeri, M., Maheras, P., Nissen, K. M., Pavan, V., Pinto, J. G., Saaroni, H., Seubert, S., Toreti, A., Xoplaki, E., and Ziv, B.: Climate of the mediterranean: Synoptic patterns, temperature, precipitation, winds, and their extremes, The Climate of the Mediterranean Region, Elsevier, Amsterdam, 301–346, https://doi.org/10.1016/B978-0-12-416042-2.00005-7, 2012.
Urban, M. A., Nelson, D. M., Jiménez-Moreno, G., Châteauneuf, J. J., Pearson, A., and Hu, F. S.: Isotopic evidence of C4 grasses in southwestern Europe during the Early Oligocene-Middle Miocene, Geology, 38, 1091–1094, https://doi.org/10.1130/G31117.1, 2010.
Utescher, T. and Bruch, A.: Klimaentwicklung vom Oligozän – Pliozän, in: Klima- und Ökosystementwicklung im Oligozän/Miozän des Ostalpenraumes, edited by: Hemleben, C., Mosbrugger, V., Nebelsick, J. H., Bruch, A., Mühlstrasser, T., Schmiedl, G., and Utescher, T., Bericht (1998–2000) des Sonderforschungsbereiches 275 “Klimagekoppelte Prozesse in meso- und känozoischen Geoökosystemen”, Universität Tübingen 1, 141–176, 135094128X, 2000.
Westerhold, T., Marwan, N., Drury, A. J., Liebrand, D., Agnini, C., Anagnostou, E., Barnet, J. S. K., Bohaty, S. M., De Vleeschouwer, D., Florindo, F., Frederichs, T., Hodell, D. A., Holbourn, A. E., Kroon, D., Lauretano, V., Littler, K., Lourens, L. J., Lyle, M., Pälike, H., Röhl, U., Tian, J., Wilkens, R. H., Wilson, P. A., and Zachos, J. C.: An astronomically dated record of Earth's climate and its predictability over the last 66 million years, Science, 369, 1383–1388, https://doi.org/10.1126/SCIENCE.ABA6853, 2020.
Zachos, J., Pagani, H., Sloan, L., Thomas, E., and Billups, K.: Trends, rhythms, and aberrations in global climate 65 Ma to present, Science, 292, 686–693, https://doi.org/10.1126/science.1059412, 2001.
Zamanian, K., Pustovoytov, K., and Kuzyakov, Y.: Pedogenic carbonates: Forms and formation processes, Earth-Sci. Rev., 157, 1–17, https://doi.org/10.1016/j.earscirev.2016.03.003, 2016.
Zamanian, K., Lechler, A. R., Schauer, A. J., Kuzyakov, Y., and Huntington, K. W.: The δ13C, δ18O and Δ47 records in biogenic, pedogenic and geogenic carbonate types from paleosol-loess sequence and their paleoenvironmental meaning, Quaternary Res., 101, 256–272, https://doi.org/10.1017/qua.2020.109, 2021.