the Creative Commons Attribution 4.0 License.
the Creative Commons Attribution 4.0 License.
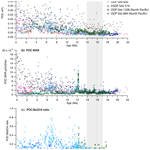
Variations in the biological pump throughout the Miocene: evidence from organic carbon burial in Pacific Ocean sediments
Annette Olivarez Lyle
The biological pump, defined as the marine biological production and sedimentation of particulate organic carbon (POC), is a fundamental process for fixing atmospheric carbon dioxide in the oceans, transferring carbon away from the atmosphere to the deep ocean, and maintaining the CO2 level of the atmosphere. The level of carbon sequestration caused by the biological pump has varied throughout the last 50 million years, from particularly weak during the warm Eocene period to much stronger during the Holocene. However, persistently warm climates from the more recent past, e.g., the Miocene Climate Optimum (MCO; 17 to 13.8 Ma – million years ago), also affected the biological sequestration of carbon. A series of scientific ocean drill sites from the equatorial Pacific exhibit very low percentages of sedimentary POC from the period prior to 14 Ma but show higher and much more variable POC percentages from the period afterwards. Although lower absolute productivity may have contributed to more limited POC burial during the MCO, higher relative POC degradation also occurred. Ratios of POC to other productivity indicators indicate a greater relative loss of POC. Temperature records suggest that higher levels of POC degradation occurred in the upper water column and that global cooling strengthened the biological pump but led to more burial variability. Similar records of low POC levels during the MCO can be found in the North Pacific, suggesting that this was a global – rather than regional – change. A weakened biological pump during warm climate intervals helps to sustain periods of global warmth.
- Article
(7413 KB) - Full-text XML
-
Supplement
(4333 KB) - BibTeX
- EndNote
The sequestration of organic carbon in pelagic sediments encompasses all biological, physical, and chemical processes – from carbon fixation via photosynthesis, water-column transport, and degradation to ultimate sea floor deposition and burial. Heterotrophic consumption, as well as inorganic oxidation, greatly reduces the total particulate organic carbon (POC) mass falling from the surface and its reactivity. POC that is eventually buried in pelagic sediments is protected from further attack because, firstly, the remainder is relatively recalcitrant after water column degradation and, secondly, POC binds to sediments, making it less accessible for further degradation (Hedges and Keil, 1995; Mayer, 1995). Buried organic matter represents a small fraction, less than 1 %, of the original primary productivity in the pelagic realm (Suess, 1980; Muller and Suess, 1979; Martin et al., 1987). In the Pleistocene pelagic equatorial Pacific, POC content found in surface sediments was typically low, around 0.2 % (Murray and Leinen, 1993), while ocean margin surface sediments contained a much higher POC content (between 1 %–2 %). The difference results from lower primary productivity in the equatorial Pacific; deeper waters (>4 km for the equatorial Pacific vs. <1 km for the ocean margins); and much slower sedimentation rates, which allowed POC to be exposed to oxygenated seawater for thousands of years.
There is evidence that during extended periods of global warmth, POC degradation tends to be greater and occurs higher up in the water column (John et al., 2014; Boscolo-Golazzo et al., 2021). Upper-water-column POC degradation results in poorer sequestration of atmospheric CO2, higher levels of atmospheric CO2 in dynamic equilibrium with the oceans, and higher nutrient levels in upwelled waters. Unlike during the last century, a period of intense global warming at the surface above cold oceans, there is not necessarily a strong oxygen minimum during stable warm periods. Long periods of warming deepen the permanent thermocline and weaken the pycnocline, allowing for more extensive mixing of oxygen into the ocean, in contrast to the modern condition, where surface warming strengthens the temperature and density contrast between the mixed layer and colder, deeper waters. The resulting strong pycnocline in the modern ocean restricts mixing and oxygen transport downward. Higher levels of dissolved oxygen and higher water temperatures found in long warm intervals thus intensify POC degradation in surface waters and reduce burial rates. Nutrients and CO2 resulting from POC degradation cycle back to the surface faster under warm Earth conditions. Surface water that is subducted to shallow depths often reappears at the surface within decades, rather than centuries, as is the case for waters that are cycled to abyssal depths. Therefore, the biological pump weakens during longer intervals of global warming (Boyd, 2015). Indeed, a recent paper by Li et al. (2023) estimated the global POC burial for the Neogene and found much less POC burial than expected for the Miocene Climate Optimum (MCO) interval.
The hypothesis that POC degradation occurred at much shallower depths in the water column during warm ocean conditions is supported by John et al. (2014). They calculated ocean depth gradients in carbon isotopes measured on planktonic foraminifera and compared them to Earth system model representations for the Paleocene–Eocene Thermal Maximum (PETM) and the greenhouse Eocene period, concluding that the Eocene experienced shallower POC degradation and recycling. Similarly, Boscolo-Galazzo et al. (2021) examined changes in the abundance of planktonic foraminifera living at different depths in the ocean during the period from 15 to 0 Ma and used stable-carbon-isotope values from foraminiferal tests, combined with Earth system models, to monitor changes in carbon flux throughout the water column. When combined with the modeling of temperature-dependent POC degradation, their results were consistent with cooling causing more particulate POC rain to penetrate deeper into the water column, leading to a stronger biological pump.
The biological pump can also be studied by examining the POC buried in the sediments. The POC mass accumulation rate (MAR; burial flux) depends upon all the processes in the water column occurring above the sediments and is the inverse of measurements that could be made in the water column because POC does not originate from the sediments below. It is a measure of the integrated effectiveness of the biological pump. While high primary productivity increases the flow of particulate POC to the sea floor, increases in heterotrophic consumption by means of zooplankton and microbes reduce the resulting downward flux. Due to the fundamental temperature dependence of metabolic activity (see, e.g., Brown et al., 2004), higher water temperatures increase the rate of POC degradation through zooplankton and microbes and decrease POC burial (e.g., Boscolo-Galazzo et al., 2018).
Preservation of POC in sediments can also be studied by comparing it to other paleoproductivity measures that are better preserved. For example, biogenic barium (bio-Ba) deposition is strongly correlated with POC rain from the euphotic zone (Dymond and Collier, 1996) and is better and more consistently preserved at the sea floor under typical oxygenated conditions (Dehairs et al., 1980; Dymond et al., 1992; Ganeshram et al., 2003). Lyle and Baldauf (2015) studied relative CaCO3 dissolution in this way, using ratios of CaCO3 to Ba. Under warm Earth conditions associated with early Cenozoic greenhouse conditions (Olivarez Lyle and Lyle, 2005, 2006; Lyle et al., 2005), percentages of buried POC were extremely low (with a Eocene average of 0.03 %). Olivarez Lyle and Lyle (2005, 2006) used bio-Ba burial to estimate an expected POC MAR, assuming modern conditions. All POC burial during the Eocene was significantly reduced compared to modern POC burial relative to production, indicating a much higher heterotrophic consumption of POC within the water column. The ratio of POC to Ba is thus an indicator of relative POC preservation.
We produced long-term, low-resolution POC and CaCO3 data, extending into the Miocene, between 2008 and 2013 for drill sites in the Pacific Ocean (Fig. 1; Table 1). In this paper, we report discrete POC measurements that we made at three scientific drill sites in the eastern and central equatorial Pacific (Ocean Drilling Program (ODP) Site 574 and Integrated Ocean Drilling Program (IODP) Sites U1337 and U1338) and combine them with data from the Ontong Java Plateau, located in the western equatorial Pacific (ODP Sites 806 and 807; Stax and Stein, 1993). We also report briefly on two sites from the northwestern Pacific (Sites 884 and 1208) to show that changes in POC burial were not limited to the equatorial region. We use these data to study changes in the biological carbon pump from the MCO to the present by comparing the patterns of POC preservation over time and, where data are available, by comparing how POC survives relative to bio-Ba, a better-preserved paleoproductivity indicator.
Table 1Locations of the drill sites discussed in this paper and their paleo-locations throughout the Miocene. Positive latitudinal (longitudinal) coordinates indicate northern (eastern) positions. Eq crossing: Equator crossing.

Li et al. (2023) used a global set of mass accumulation rates to show that there was a global low in POC MARs during the MCO, with the primary objective of the paper being to test the Monterey hypothesis, which posits that high levels of δ13C occurring between 17 and 13 Ma resulted from increased POC burial. They found that the MCO interval exhibited globally reduced POC burial and was not the cause of high δ13C levels at that time. The objective of this paper is different. We chose to examine a focused region (the equatorial Pacific) and use additional sedimentary data to separate changes in production from changes in preservation in the ultimate POC record. We examine four hypotheses for the limited POC burial during the MCO – (1) low primary productivity, (2) POC degradation in warmer deep waters, (3) POC degradation in warmer surface waters, and (4) a fundamental change in the proxy–POC relationship. We find that the low POC MARs in the equatorial Pacific largely result from better preservation of sedimentary POC as the Earth cooled, due to reduced degradation in the surface ocean.
We measured over 1600 samples (1215 presented here plus 388 previously published) of POC and CaCO3 from five sites of the Deep Sea Drilling Project, the Ocean Drilling Program, and the Integrated Ocean Drilling Program (Sites 574, 884, 1208, U1337, and U1338; see Fig. 1 and the tables in the Supplement). Freeze-dried sediment samples were analyzed using coulometry and the furnace methodologies presented in Lyle et al. (2000), which provide accurate POC values at very low levels.
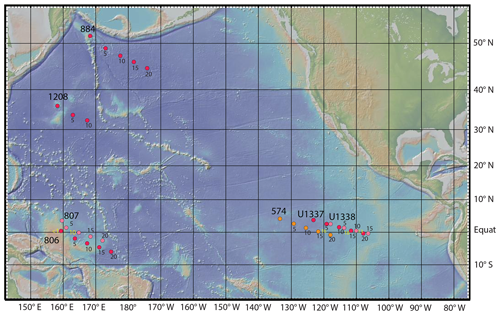
Figure 1Drill site locations discussed in this paper. The paleo-locations for each site are shown at 5 Myr intervals using a fixed-hotspot rotation pole for the Pacific Tectonic Plate. The equatorial ODP drill sites (Sites 806 and 807) were drilled during ODP Leg 130 (Stax and Stein, 1993). Sites U1337 and U1338 were drilled during IODP Expedition 321 (Lyle et al., 2019; Pälike et al., 2010). Site 574 was drilled during DSDP Leg 85 (Mayer et al., 1985). Two other sites are briefly discussed – Site 1208, located on Shatsky Rise (Bralower et al., 2002), and Site 884, near Detroit Seamount, located at the northern end of the Hawaiian–Emperor seamount chain (Rea et al., 1993). Yellow numbers indicate the ages of the sites' Equator crossings, each corresponding to a local peak in primary productivity and sediment deposition. The base map was made with GeoMapApp (https://www.geomapapp.org/, last access: 1 September 2024) (Ryan et al., 2009). Equat: Equator.
First, the total carbon of the sediment sample was measured through combustion in a 1000 °C furnace and analyzed using a coulometer. POC was determined by acidifying a second, larger aliquot of the sample to remove the CaCO3-based CO2, before analyzing the remaining sediment residue via combustion in pure oxygen at 1000 °C using coulometry. From these two measurements, the CaCO3 fraction was calculated based on the difference between the CO2 measured for total carbon and the organic carbon fraction. We found that this method is accurate for estimating CaCO3 down to the <1 % level and for estimating POC at the 0.01 % level (Olivarez Lyle and Lyle, 2005). We checked for consistency in our carbon analyses, as described in Olivarez Lyle and Lyle (2005), by running an in-house sediment standard, “Midway”, with each carbon run; hundreds of analyses and summary statistics have been compiled for the Midway standard, exhibiting a total carbon value of 2.64±0.02 wt % (n=523) and a POC value of 0.85±0.01 (n=570). We also repeated the analysis of every fourth unknown sample during each run day. We monitored both total carbon and organic carbon. The average absolute difference between repeated unknown samples of POC was <0.01 wt %, and if this difference exceeded 0.02 wt %, we reanalyzed the sample. Data for Site 574 sediments older than 12 Ma are reported in Piela et al. (2012). Site 574 POC and CaCO3 data spanning the years from 12 to 2 Ma were analyzed later in our laboratory and are published here. Regarding Sites U1337 and U1338, POC data are reported in Wilson (2014; PhD thesis), though they are not discussed in detail within the thesis.
POC and CaCO3 data from Sites 884 and 1208 are also included. All the data for Site 1208 were analyzed in our laboratory using the same analytical methods as those described above. Site 884 exhibits a mixture of our data and culled shipboard carbon data, as marked in Table S8 in the Supplement. We included the shipboard data for depth intervals not analyzed in our laboratory, primarily those younger than 4.8 Ma. Typically, we do not use shipboard POC data from the Deep Sea Drilling Project (DSDP) or the ODP because their POC determinations are calculations, not measurements, based on the difference between measured total carbon and measured CaCO3. This is inaccurate for POC levels below 0.3 % in the presence of significant (>15 wt %) sedimentary CaCO3 (Olivarez Lyle and Lyle, 2005). Hence, we only used shipboard POC and CaCO3 data from Site 884 (Table S8) when CaCO3 levels were less than 15 wt %. The error in POC when using the shipboard method at this level of CaCO3 is relatively small.
We include data from Stax and Stein (1993) for Sites 806 and 807. They measured POC by dissolving an unweighed split of the sample with HCl and measuring the resulting POC after dissolving the CaCO3. They then corrected the split POC to the POC of the total sample by measuring the total carbon on a separate split of the sample and calculating the relative proportion of POC. Using this method, they could dissolve a much larger split of sediment containing POC, allowing them to be accurate when using a low POC content while also enabling them to measure other POC properties.
The net deposition and preservation of POC at Sites U1337, U1338, and 574 are estimated from ratios to the barium content in the sediments. Extensive work has shown that barium is precipitated in the water column as BaSO4 via organic matter oxidation; is well preserved; and, importantly, is a good proxy for primary productivity (Dymond et al., 1992; Dymond and Collier, 1996; Ganeshram et al., 2003). Although barium sulfate can form through other processes, in equatorial Pacific sediments, the Ba content is primarily biogenic in origin and not diluted by other Ba-containing components, such as barium in terrigenous clays (Piela et al., 2012; Lyle and Baldauf, 2015). We calibrated Ba measurements from normalized median-scaled (NMS) X-ray fluorescence (XRF; see Lyle et al., 2012) against discrete ICP-MS (inductively coupled plasma mass spectrometry) analyses for Sites U1337 and U1338 (Wilson, 2014). For Site 574, we used ICP-MS barium data from Piela et al. (2012) for sediments older than 12 Ma.
We used XRF-estimated total BaSO4 to calculate ratios to other biogenic elements in order to investigate POC preservation. We did not partition total barium to remove non-biogenic sources because the equatorial Pacific sediments at Sites U1337 and U1338 primarily consist of biogenic remains and contain minimal clay minerals (Lyle and Baldauf, 2015), meaning the majority of the barium is biogenic in origin. We further tested this assumption by making normative estimates of terrigenous clay-fixed Ba and authigenic oxide-fixed Ba from Site U1338, using other XRF-measured elements (Al, Si, Ca, Mn, Fe, and Ti) as well as discrete opal calibrations (Lyle and Baldauf, 2015). Assigning typical Ba content to the normative sedimentary components, we found that biogenic BaSO4 accounted for an average of 93 % ± 4 % of the total BaSO4 amount. The greatest potential error was found in sections with the highest clay content, i.e., in the younger sediments of each site. In these sections, the POC : BaSO4 ratio was highest, and the estimated POC preservation was best. Any bias based on total BaSO4 would have likely reduced the ratio and apparent POC preservation. Therefore, the total-BaSO4 method we used is conservative.
For all sites, we calculated POC mass accumulation rates (MARs), equivalent to the POC burial flux, as another indicator of the rate of POC deposition and burial. MARs have units of mass per unit area per unit time and are typically reported in g (cm2×103 yr)−1. For any component in a sedimentary mixture, calculating the mass accumulation rate eliminates artifacts in the resulting data profile over depth or time that are inevitably caused by the variable deposition of the other sedimentary components. Converting weight percentage data to MARs is particularly important for evaluating changes in minor components, such as POC, because changes in the deposition of major components can dominate and distort actual changes in the component of interest. However, MAR calculations are subject to errors (such as those arising from sedimentation rates), primarily caused by an imprecise age model. This issue is addressed below.
We developed age models for all sites to calculate MARs and further study rates of burial. See the Supplement for details and for data from each of the sites. MARs are developed by making an age model (an age-versus-depth profile), which is differentiated to calculate a sedimentation rate (depth of sediment deposited per unit time; cm kyr−1) over the time interval of interest. The sedimentation rate, multiplied by the dry bulk density, results in a bulk MAR (grams of solid material per cm2 kyr−1) for the sediment over a given age interval. The MARs for individual components are calculated by multiplying the bulk MAR by the weight fraction (the weight percentage divided by 100) of the component in the dry sample.
3.1 Sites U1337 and U1338
For Site U1337 (24 Myr old crust), we used an age model astrochronologically tuned to 20 Ma by incorporating the Drury et al. (2017, 2018) age model up to 8.2 Ma and by correlating the 20 Myr combined isotope record from Site U1337 (Holbourn et al., 2015; Tian et al., 2018) with the stable-isotope stack from Westerhold et al. (2020) up to 20 Ma. For Site U1338 (18 Myr old crust), we used the established age model up to 8.2 Ma (Lyle et al., 2019). The Site U1338 ages greater than 8.2 Ma were derived by correlating the CaCO3 profile from Site U1338 with that from Site U1337, which is justified because CaCO3 profiles are very similar across the central and eastern Pacific (Lyle et al., 2019; Mayer, 1991).
3.2 Site 574
Site 74 (35 Myr old crust) was drilled in 1985 during Leg 85 of the Deep Sea Drilling Project and lies about 1000 km west of Site U1337. It was one of the first sites to have sediments cored with hydraulic piston corers. We performed XRF scanning on the upper five cores of Holes 574 and 574A and used these data, along with the gamma ray attenuation (GRA) bulk density record from deeper sections, to make a new splice up to ∼17.4 Ma (with a 225 m composite depth (the base of Holes 574 and 574A)), using the Code for Ocean Drilling Data (CODD) software (https://www.codd-home.net/, last access: 8 August 2023). The new splice was then correlated with Site U1337 via a CaCO3 profile produced using the Site 574 GRA bulk density to estimate the CaCO3 content. In the eastern and central Pacific Ocean, the GRA bulk density is highly correlated with changes in CaCO3 content (Mayer, 1991). The non-carbonate fraction in the equatorial Pacific primarily consists of low-density, high-porosity biogenic silica remains from diatoms. The GRA bulk density data also have a much higher resolution than the discrete carbonate analyses used for calibration. The spliced GRA density record from Site 574, along with the assigned ages (Table S2) and the new splice developed for Site 574 (Table S1), can be found in the Supplement.
3.3 Sites 806 and 807
We used polynomial fits to ages updated to the biostratigraphic ages used for Sites U1337 and U1338 (Expedition 320/321 Scientists, 2010a), applying them to the biostratigraphic datum levels for Sites 806 and 807, as reported in the Initial Reports volume (Kroenke et al., 1991). Such fits are less accurate than a direct correlation, but these sites do not have long stable-isotope records, and it was not possible to correlate the carbonate records with the eastern Pacific. While not as accurate as the other age models, these ages should still be accurate to within ±0.2 Ma or better. The major potential error is the spacing of biostratigraphic sampling, which is approximately every 3 m for foraminifera and every 4.5 m for calcareous nannofossils (Chaisson and Leckie, 1993; Takayama, 1993).
3.4 Sites 1208 and 884
Age–depth profiles for Site 1208 (Shatsky Rise (up to 12.4 Ma)) and Site 884 (Detroit Seamount, located at the northern end of the Hawaiian–Emperor seamount chain (up to 19.7 Ma)) were made using linear interpolations between magnetochrons. Magnetochrons were determined via shipboard pass-through magnetometry, with further analysis being shore-based. Shipboard magnetic measurements were made every 10 cm at Site 884 and every 5 cm at Site 1208. Magnetochrons for Site 1208 were taken from Evans (2006), and shipboard paleomagnetic data were used for Site 884 (Rea et al., 1993). All magnetochrons have updated ages based on Westerhold et al. (2020). The accuracy of the ages should be within 0.1 Ma. The profiles are listed in the Supplement. Site 1208 was located on Shatsky Rise itself and experienced an extreme slowdown in sedimentation rate from the middle Miocene through the Late Cretaceous, which may have affected the early part of the profile.
The bulk MAR for each site was calculated as the product of the sedimentation rate (from the age–depth profiles) and dry bulk density to yield the total mass of sediment deposited over a given age interval (the bulk MAR). Dry bulk density was estimated by correlating discrete data on physical properties from each drill site with the reported GRA data on wet bulk density. We used this correlation to develop a higher-resolution profile of dry bulk density from the GRA data.
4.1 Organic carbon variations
There is a striking difference between the POC records from the Pacific equatorial sites older than ∼13 Ma and those from the younger sections, as illustrated in Fig. 2. Low percentages of sedimentary POC, POC MARs, and POC : BaSO4 increased after the MCO, resulting in higher and more scattered values between 14 and 12 Ma. POC weight percentages were much lower in the older record compared to those of the Pliocene and Pleistocene (Fig. 2a). During the MCO, between 17 and 14 Ma, POC weight percentages at these five sites averaged 0.043±0.014 %, in contrast to the nearly 3-fold increase (0.124±0.058 %) occurring in the Pleistocene and Pliocene (0–4 Ma), and we note that the MCO values are more similar to the average values measured for the Eocene equatorial Pacific (with POC roughly corresponding to 0.03 wt % (>34 Ma); Olivarez Lyle and Lyle, 2006). Both the concentration of sedimentary POC and its variability were much lower in the period prior to 14 Ma.
High dilution caused by other sedimentary components can lower POC percentages, but dilution in the equatorial Pacific requires the higher preservation and burial of either biogenic CaCO3 or biogenic SiO2 relative to modern conditions. Nonetheless, this possibility can be evaluated by calculating the POC MAR, which removes the dilution effect and provides a measure of the actual burial flux. Figure 2b shows the POC MAR data for the five equatorial Pacific sites. We note a relatively low and consistent POC MAR between 18 and 14 Ma. Somewhat higher POC MARs occur prior to 19 Ma, with higher but very scattered MARs observed after 14 Ma.
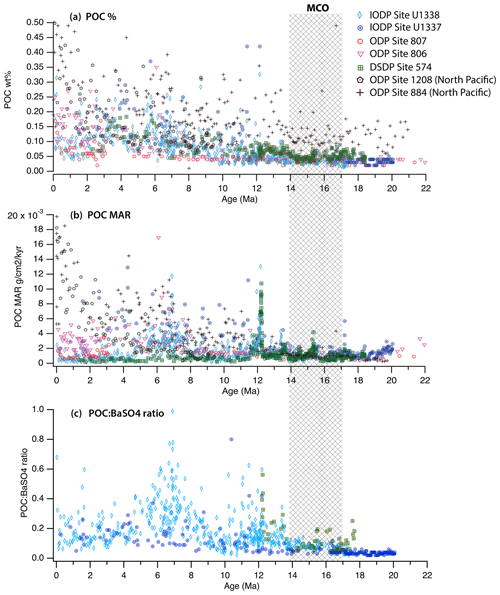
Figure 2POC percentages (a) and POC MARs (b) for the equatorial drill sites (Sites 574, 806, 807, U1337, and U338) and the North Pacific sites (Sites 1208 and 884). POC : BaSO4 ratios (c) are only available from Sites 574, U1337, and U1338. These records show evidence of low POC content and poor POC preservation during the Miocene Climate Optimum (MCO; 17–13.8 Ma) and the early Miocene.
4.2 Variations in biogenic barium
Ba was measured at three of the sites, all located in the high-productivity region of the eastern Pacific. The POC : BaSO4 ratio compares the burial of more labile POC (<1 % POC preserved) to that of better-preserved biogenic Ba (∼30 % preservation; Dymond et al., 1992; Dymond and Lyle, 1994). Since Ba has a linear relationship with POC in the particulate rain of the modern equatorial Pacific Ocean (Dymond and Collier, 1996), changes in the POC : BaSO4 ratio are interpreted as reflecting the relative preservation of POC. Figure 2c shows that there was reduced POC burial relative to Ba prior to 14 Ma compared to later in the records.
Ba was measured at Site 574 using ICP-MS for the sediment column older than 12 Ma (Piela et al., 2012). At Sites U1337 and U1338, Ba was measured by scanning XRF throughout the entire composite sediment column (Lyle et al., 2012; Wilson, 2014; Lyle and Baldauf, 2015). All sites had low POC : BaSO4 ratios (i.e., poor POC preservation) prior to 14 Ma during the MCO and exhibited higher ratios with much greater variation afterwards.
Low POC percentages during the MCO were not only confined to the equatorial Pacific but were also found in the north Pacific. We also analyzed two sites in the North Pacific that showed increased POC burial as the Miocene progressed. At Site 1208 on Shatsky Rise and Site 884 on Detroit Seamount (Fig. 1), POC MARs have increased strongly since the MCO (Fig. 2a and b; Tables S8 and S10). A straightforward interpretation of these records, however, is complicated by the fact that there was a major increase in the sedimentation rate at Site 1208 after a Paleocene hiatus, which in itself also caused increased POC MARs after 7.5 Ma. As such, it is unclear to what extent the local sedimentation regime played a role in the increase in POC MARs relative to regional levels of preservation. At Site 884, sedimentation rates increased in the early Miocene, leading to higher POC MARs after the MCO (see Fig. S5). There is no reason to suspect that the Site 884 record was strongly influenced by changes in the local sedimentary environment; however, there is overall much higher clay deposition at this site, which might have affected POC burial. In summary, these two sites suggest that there was a general increase in POC MARs throughout the Pacific after the MCO, but more records need to be produced and evaluated to support this hypothesis. However, it is important to highlight data from a recent study by Li et al. (2023), who found surprisingly limited POC burial during the MCO worldwide, supporting the hypothesis that a global slowdown in POC deposition occurred during the MCO.
Another important observation is that periods that have been identified previously as high-productivity phases in the late Miocene can also exhibit an increased relative burial of POC. This effect is most obvious at Site U1338, an eastern equatorial Pacific drill site (Figs. 2 and 3). The Late Miocene Biogenic Bloom (LMBB) had elevated POC MARs and was a period of elevated POC : BaSO4 ratios. Elevated POC MARs during the LMBB were also exhibited at Sites 806, 807, and 884. Similarly, there were significant POC MARs at around 12 Ma in an earlier interval, which appear to be productivity-related (Lyle and Baldauf, 2015). Not only are such intervals high in POC deposition, but they are also high with respect to POC : BaSO4. It appears that under certain conditions of high productivity, both the burial and preservation of POC are enhanced, as indicated by the POC : BaSO4 ratios. How apparent elevated productivity affects ultimate POC burial deserves further study.
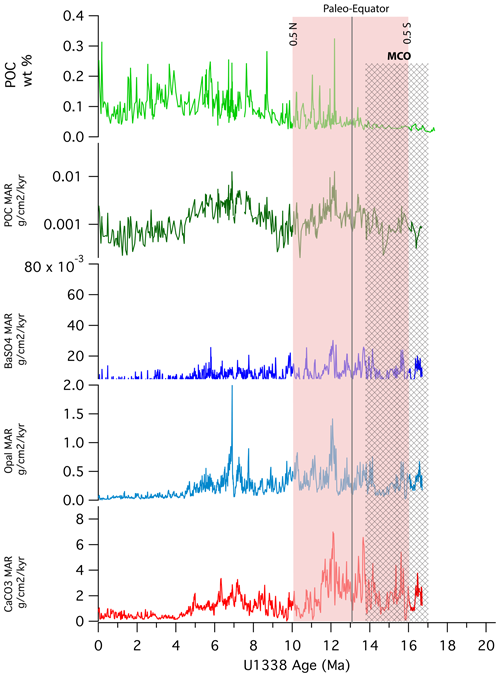
Figure 3Time series of (from top to bottom) POC weight percentages, POC MARs, BaSO4 MARs, biogenic SiO2 MARs, and CaCO3 MARs for Site U1338, located on ocean crust formed at 18 Ma. High biogenic MARs are characteristic of later high-productivity intervals. However, the MCO exhibits low POC content and a low POC MAR, despite Site U1338 being relatively near the Equator during this interval. The paleo-Equator line marks the time when plate tectonic movement aligned the site with the Equator.
We expect variability in the deposition of biogenic particulate matter resulting from large-scale tectonic–biogeographic processes and from global intervals of high productivity, as well as from regional variation in primary productivity. We note that POC depositional variability from 12 to 0 Ma is high, ranging from 0.03 wt % to 0.40 wt %. The variation is caused partly by the geographic position of the site relative to the high-equatorial-productivity zone and partly by the presence of high-productivity intervals since the MCO. Some variation may also result from the protection of POC by the deposition of other sedimentary components. Nevertheless, we note that more slowly accumulating sediments from the Pliocene and Pleistocene tend to have high POC percentages (Figs. 3 and 4) but do not correspond to high biogenic MARs. In other words, factors other than high productivity caused the better preservation of POC between 8 and 0 Ma since high POC percentages are not necessarily associated with other indicators of high paleoproductivity. All records from the equatorial Pacific show evidence of low POC percentages and POC MARs during the MCO (Fig. 2), while sediments from the Pliocene and Holocene have higher POC percentages, even though many of the sites exhibit low POC MARs.
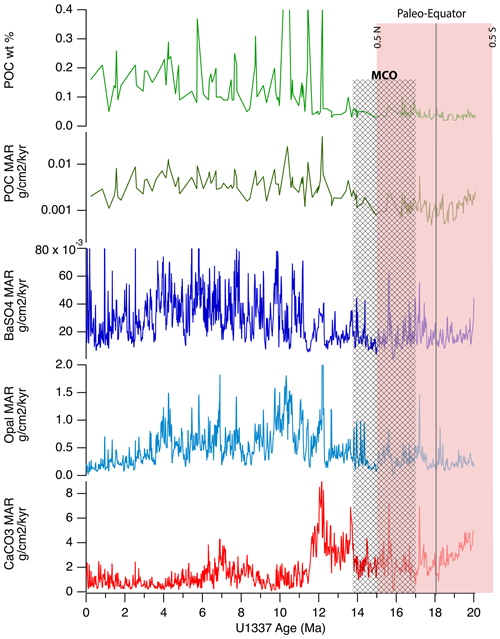
Figure 4Time series of (from top to bottom) POC weight percentages, POC MARs, BaSO4 MARs, biogenic SiO2 MARs, and CaCO3 MARs for Site U1337, located to the west of Site U1338 on 24 Myr old ocean crust. The time series of biogenic MARs are more complex here because of sediment focusing in the younger part of the record. However, the MCO exhibits low POC content and a low POC MAR, despite Site U1337 being relatively near the Equator during this interval. The paleo-Equator marks the time when plate tectonic movement aligned the site with the Equator.
We investigated the possibility that bioturbation might bias the POC records since POC has remained in the equatorial Pacific's 5–10 cm sediment mixed layer for thousands of years (Kadko and Heath, 1984; Broecker et al., 1991). It is possible that a change in sedimentation rate from fast to slow might enhance POC degradation by enhancing exposure to oxygenated bottom waters. We know that labile POC is degraded rapidly within the equatorial Pacific sediment mixed layer (Stephens et al., 1997), so perhaps more recalcitrant POC would be degraded if sedimentation rates slowed. If this were true, there would be a correlation between the sedimentation rate and POC content since high sedimentation rates would shorten the time that POC spends in the mixed layer. We checked this and found no correlation.
We interpret the scatter of POC percentages as reflecting the localized response to the timing of productivity drivers changing the transport of POC from surface waters and the better preservation of POC in more recent times. There are two major productivity drivers in the equatorial Pacific: first, the movement of the site across the equatorial high-productivity region caused by the tectonic motion of the Pacific Plate (the line of high productivity for the Neogene; Lyle, 2003; Moore et al., 2004) and, second, the occurrence of high-productivity intervals, which typically are limited in both space and time. A well-documented global productivity interval occurred between 8 and 4.5 Ma, known globally as the Late Miocene Biogenic Bloom (LMBB) (Dickens and Owen, 1999; Diester-Haas et al., 2005; Lyle and Baldauf, 2015; Drury et al., 2017; Lyle et al., 2019; Karatsolis et al., 2022; Gastaldello et al., 2023). An earlier high-productivity interval has been identified as having occurred in the eastern equatorial Pacific between 13 and 10.5 Ma (Lyle and Baldauf, 2015), extending to ∼14 Ma (Holbourn et al., 2014).
5.1 Tectonic passage through the equatorial-productivity zone and equatorial primary productivity
Modern studies of equatorial biological productivity, including direct measurements obtained in the water column, MARs in surface sediments, and productivity based on interpretations of satellite color, find that the particulate flux, both at the surface and to sediments, is highest at the Equator and strongly decreases to the north and south (Wyrtki, 1981; Chavez and Barber, 1987; Dugdale et al., 1992; Murray and Leinen, 1993; Honjo et al., 1995; Behrenfeld et al., 2005). This pattern of high equatorial biogenic flux has been found to have occurred throughout the Neogene (Moore et al., 2004; Berger, 1973). The records provide strong evidence that the equatorial divergence and upwelling driven by the southeastern trade winds crossing the Equator were persistent features of the Cenozoic oceans, causing high primary productivity at the Equator. The magnitude of equatorial productivity has not remained constant over time; instead, it has waxed and waned along with global climate change. We expect to find an increase in the productivity and deposition of biogenic sediments as the movement of the Pacific Plate brings a drill site into position at the Equator, and we expect to find a decrease in biogenic MARs as the site moves away from the Equator.
For example, Piela et al. (2012) found that a high biogenic silica mass accumulation rate (biogenic silica MAR) and a high barium mass accumulation rate (Ba MAR) occurred during the Site 574 paleo-Equator crossing at 16.25 Ma, despite low POC MARs and low CaCO3 MARs. They hypothesized that the dissolution of CaCO3 exposed more POC in surface sediments to potential degradation and reduced POC with respect to other productivity signals. It could also represent the preferential degradation of POC in surface waters.
At Site U1338, high burial rates of biogenic components other than POC coincide with the period when Site U1338 was within ±0.5° (55 km) of the paleo-Equator during the middle Miocene (16–10 Ma; Fig. 3). POC MARs, unlike the MARs of other biogenic components, were not as enhanced during the MCO. POC burial was minimized relative to that of other biogenic components during the MCO and immediately afterwards. Also, at Site U1338, the high POC MAR and opal MAR corresponding to 12 Ma are roughly equivalent to modern MARs in surface sediments, as reported by Murray and Leinen (1993). CaCO3 MARs are affected not only by high productivity but also by changes in carbonate dissolution over time. Nevertheless, much of the variation in CaCO3 at Site U1338 is consistent with variations in both opal and BaSO4 MARs, indicating a strong productivity signal at the site.
The 15–20 Ma section of the Site U1337 oxygen isotope record is part of the Cenozoic oxygen isotope splice from Westerhold et al. (2020) and, thus, along with Site U1338, represents the MCO interval of the equatorial Pacific. The sediments from Site U1337 (Fig. 4) crossed the Equator from southeast to northwest at an earlier time than Site U1338 did (21 to 15 Ma vs. 16 to 10 Ma, respectively) and had relatively low biogenic MARs in the older sediments compared to those found later in the U1337 section. This is in part caused by sediment focusing within the younger part of the record, especially in the intervals 6.2–5.4 and 4.5–3 Ma (Lyle et al., 2019, and the associated supplemental material). The sediment focusing is indicated by anomalously high Pleistocene and Pliocene sedimentation rates compared to those at piston cores and other sites at this latitude. Furthermore, erosional channels to the northeast of the site were found in the site survey (Fig. 5; Moore et al., 2007; Lyle et al., 2019). Nevertheless, sediment focusing did not affect the time interval spanning the MCO, for which we find very low POC percentages and POC MARs. Such low values contrast with the expected pattern of higher biogenic sedimentation beneath the high-productivity region of the paleo-Equator. We also note that BaSO4 MARs at Site 1337 were 3 times higher than those at Site 1338 during the MCO, implying greater primary productivity and organic carbon production, yet a POC signal was not preserved in the sediments.
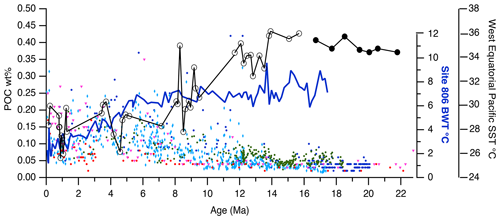
Figure 5POC weight percentage time series for the equatorial Pacific (left axis), along with a bottom water temperature (BWT) record from Site 806 (Lear et al., 2015) and a western equatorial Pacific sea surface temperature (SST) record (Mg / Ca proxies), where unfilled circles indicate Site 806 and filled circles indicate Site 872 (Guillermic et al., 2022), with Site 872 data obtained from Sosdian et al. (2018). BWT in the Pacific did not change much until after 6 Ma, which was too late to affect POC degradation during the MCO, while SST remained high until the end of the MCO. SST dropped by about 3 °C at the end of the MCO, followed by another ∼3 °C drop by 9 Ma.
Sites 806 and 807, located in the western Pacific on the Ontong Java Plateau, crossed the equatorial region after 1 Ma and at 10 Ma, respectively. In the modern ocean, the upwelling signal in the far western equatorial Pacific is not nearly as strong as that in the eastern equatorial Pacific (Behrenfeld et al., 2005; Rousseaux and Gregg, 2017). Site 806 exhibits its highest POC MAR in the Pleistocene, as expected due to its recent Equator crossing. However, Site 807 shows little sign of its Equator crossing in its POC MARs at 10 Ma, although both it and Site 806 exhibit POC MARs resulting from the LMBB. However, both sites exhibit low POC MARs during the warm MCO.
5.2 Past high-productivity intervals
Figure 3 shows the time series of all biogenic MARs observed at Site U1338. All biogenic MARs are elevated between ∼14.5 and 11.5 Ma, indicating high primary production despite the limited POC burial during the MCO. High productivity around 14 Ma at Site U1338 was first noted by Holbourn et al. (2014), who suggested that biogenic silica production was a factor in ending the MCO by reducing high atmospheric CO2 levels.
Both episodes are clearly observed at Site U1338. At Site U1337, the biogenic Si MARs are similar to those at Site U1338 during the older interval, but there is another interval, filled with laminated diatom mats between 10 and 12 Ma (200–250 m composite coring depth below sea floor), that might represent accumulation near the subduction boundary between the South Equatorial Current and North Equatorial Countercurrent (Expeditions 320/321 Scientists, 2010b). So, at Site U1337, the two separate higher-productivity intervals are joined by a third interval of higher deposition. Both Site 806 and Site 807 show elevated POC MARs associated with the LMBB, indicating that these global high-productivity intervals affected all sites in the equatorial Pacific.
5.3 Four hypotheses for explaining the limited POC burial during the MCO
We propose four working hypotheses for the cause of the low levels of POC MARs along the Pacific Equator during the MCO: (1) generally low primary productivity during the MCO; (2) warmer deep waters and increased POC degradation in the lower water column or at the sediment surface; (3) increased degradation of POC in surface waters; and (4) low apparent POC levels as an artifact of changing proxy relationships, e.g., Ba fixation in relation to POC fixation in particles. Only in hypothesis 1 (low primary productivity) does the relationship between POC production, the production of productivity proxies, and the proxies' ultimate burial remain the same; the other hypotheses assume that a change in these relationships occurred during the MCO.
5.3.1 Hypothesis 1: low productivity is the primary factor for low POC levels during the MCO
Under hypothesis 1, the observed low POC percentage during the MCO reflects the low average productivity during the MCO interval. However, the data do not support this hypothesis. Each of the sites was in a different position relative to the equatorial high-productivity zone at the time of the MCO. The sites near the Equator had POC percentages and POC MARs as low as those farther away. Furthermore, there is no evidence of global low productivity during the MCO. Site U1338, which was in the equatorial zone toward the end of the MCO, exhibited increases in POC percentage and POC MAR at the end of the MCO. Since other paleoproductivity indicators tended to be high during the MCO, it is apparent that low POC MARs are not a result of low MCO primary productivity.
In summary, while we find some evidence of somewhat lower productivity during the MCO, high primary productivity associated with drill site Equator crossings is reflected in the increased burial of other biogenic sediment components and not in that of POC. Therefore, we conclude that additional factors caused the lower POC MARs during the MCO.
5.3.2 Hypothesis 2: warmer deep waters and more POC degradation in the lower water column or at the sediment surface led to low POC MARs during the MCO
Particulate matter falls through the oceans at a rate of ∼50–150 m d−1 once it leaves the surface ocean (Honjo et al., 1982; Berelson, 2001; McDonnell and Buessler, 2010), thus spending 7–10 d above 1000 m in the upper water column. Particles transiting the remaining 3 km of the water column (assuming an average ocean water depth of 4 km) require an additional 3 weeks to a month before reaching the bottom. Afterwards, particulate organic carbon (POC) resides at the sediment surface for decades to centuries before final burial. Provided the POC has survived its passage through the upper water column, it is possible that temperatures or other processes in the lower water column have controlled the burial of POC through the temperature-dependent degradation of POC. If the change in POC degradation occurs primarily in the lower water column, one consequence is that the biological pump would still function, albeit at a somewhat lower rate.
There are two problems with the hypothesis concerning the lower-water-column control of POC burial. First, most POC is remineralized in the upper water column in the modern oceans, meaning only the more recalcitrant or protected POC fractions survive to reach abyssal depths. Second, note that cooling of the abyssal Pacific occurs much later than the end of the MCO (Fig. 5); thus, any temperature-linked degradation of POC in relatively warm deep waters should decrease after the deep waters cool. As Fig. 5 illustrates, Pacific deep waters were only slightly warmer during the MCO compared to immediately after this period and stayed at a relatively constant temperature of 7 °C until about 6 Ma (Lear et al., 2015). Only after 6 Ma did the deep waters cool to a modern temperature of about 2 °C. Therefore, we expect a POC signal primarily caused by deep-water cooling to have a very different signal from the one observed.
The abyssal temperature change during the MCO was relatively small. Data from Lear et al. (2015) show that the MCO was 0.4 °C warmer than the next 3 Myr of the temperature record at Site 806 (Fig. 5). Kochann et al. (2017), in contrast, described abyssal temperature changes of 1.7° at the end of the MCO at Site U1338 and 2.2 °C at Site 1146 in the South China Sea. However, they did not observe consistently warm temperatures throughout the intervals in the MCO that they measured, in contrast to the consistently low POC content. Kochann et al. (2017) noted a transient 2.6 °C warming of bottom water temperatures at the onset of peak MCO warmth (15.5 Ma) at Sites U1337 and U1338. However, both Lear et al. (2015) and Kochann et al. (2017) found intermittent warming during the MCO, which is inconsistent with abyssal warmth being a driver of low POC throughout the entire MCO.
A low percentage of particulate matter derived from total productivity reaches the sea floor, and sediment trap studies have shown that the particulate flux that reaches the sea floor is relatively rich in POC. The deepest sediment traps in the Joint Global Ocean Flux Study (JGOFS) tropical Pacific experiment caught a particulate flux containing around 5 wt % POC (Honjo et al., 1995; using particulate rain from sediment traps between 2191 and 3618 m depth and within ±5° latitude of the Equator), which, subsequently, needed to be degraded to have the typical POC content found in surface equatorial sediments, around 0.2 % to 0.3 % (Murray and Leinen, 1993; Prahl et al., 1989). This order-of-magnitude loss of organic carbon, from the deepest waters to surface sediments, appears to reflect a consistent level of degradation in the pelagic equatorial Pacific, as also shown by the core top values of POC in the drill site data. Interestingly, the highest POC levels found in surface sediments, as measured in the JGOFS transect by Murray and Leinen (1993), occur in off-equatorial sites where clay is much more abundant and sedimentation rates are much lower. The lower equatorial POC levels are partly a dilution effect due to additional CaCO3, compensated for by higher sedimentation rates near the Equator (Murray and Leinen, 1993).
5.3.3 Hypothesis 3: warmer surface waters and more POC degradation in the upper water column during the MCO
Here, we discuss our preferred hypothesis based on the temperature dependency of the biological pump – i.e., higher POC degradation in warmer surface waters prior to and during the MCO caused reduced POC burial due to diminished rates of POC transfer to deep waters. This hypothesis requires that the converse is also true – i.e., that the cooling of surface waters following the MCO resulted in increased POC transfer to the abyss. Note that for all warm–cold and cold–warm transitions, the strength of the POC sedimentary signal that is ultimately preserved is partly dependent on the productivity regime at each drill site.
In Fig. 5, we present a sea surface temperature (SST) record for the western equatorial Pacific using Mg / Ca SST proxies from Guillermic et al. (2022), who combined Site 806 data with early Miocene data from Site 872, a tropical guyot (Sosdian et al., 2018). Sea surface temperatures in the early Miocene, prior to the MCO, were warm in the western equatorial region, ranging between 34 and 36 °C. Surface temperatures cooled quickly at the end of the MCO by about 3 °C and then cooled further by ∼3 °C around 11 Ma. The cooling SST coincided with the increase in POC in our sedimentary record. Low levels of POC in the period prior to the MCO (Fig. 2) provide further evidence that surface water warmth is an important factor in POC burial – i.e., that limited POC burial is not restricted to the MCO.
Modeling of carbon isotope distributions from foraminifera dwelling at different depths (see John et al., 2014, for details regarding the Eocene) and observations of plankton distributions suggest that POC degradation occurs primarily within the surface ocean layer, above 1000 m. Supplemental material from Boscolo-Galazzo et al. (2021) showed that there were much steeper δ13C depth gradients in older time intervals, modeling much shallower depths for POC degradation and a sharper O2 minimum than in the Holocene. Using their temperature-dependent model at Site U1338, located in the equatorial Pacific, the flux of particulate POC passing through 600 m is 3 to 4 times greater in the Holocene, relative to 15 Ma, for the same level of primary productivity. Similarly, we observe a 3–4-fold increase in POC sediment content over the same time interval at Site U1338. If this reflects degradation in the water column, a much smaller POC flux was sequestered in abyssal waters during the warm MCO climate compared to modern conditions. In other words, warm conditions caused a weaker biological pump.
In the modern ocean, however, the POC content of particulate rain that arrives at the abyssal sea floor is significantly larger than the POC content buried in surface sediments, as noted previously in sediment trap studies. The particulate rain captured in deep sediment traps within the equatorial Pacific region (±5° of the Equator) contains about 5 % POC (Table 5 of Honjo et al., 1995). This value is an order of magnitude higher than the POC concentrations found in surface sediments, which range between 0.23 % and 0.33 % (Murray and Leinen, 1993). Lower POC MARs away from the Equator reflect the lower particulate rain rates away from the Equator, not the lower POC content in particulate rain. Differences between CaCO3 rain and biogenic SiO2 (bio-SiO2) rain were observed but did not strongly affect the total POC preserved. During the Holocene, productivity apparently affected the rate of deposition of particulate rain but did not influence its composition as much. We should be mindful of the role that the upper water column plays in determining both the magnitude of the biological pump and the level of POC content in sediments.
We note that for the period after the MCO, our data show an overall increase in the ratio of POC to BaSO4, particularly during periods previously identified as high-productivity events. For example, at Site U1338 (Fig. 2c), where we have the best record, the POC : BaSO4 ratio is greatest during the Late Miocene Biogenic Bloom (Dickens and Owen, 1999; Diester-Haas et al., 2005; Lyle and Baldauf, 2015; Drury et al., 2017; Lyle et al., 2019; Karatsolis et al., 2022).
5.3.4 Hypothesis 4: a change in proxy relationships for productivity changes estimates of paleoproductivity
Another hypothesis worth considering is that during the MCO, there was a change in the response of the proxy compared to the expected modern response. For example, diatom deposition or POC : BaSO4 might have behaved differently with respect to POC production. Under these conditions, there may have been lower actual POC export to the interior ocean than indicated by the proxy, minimizing the deposition of POC without indicating lower productivity. We believe that there is some likelihood that the relationship between the export of particulate POC from the euphotic zone and other biogenic components may be somewhat different under warm Earth conditions, but we propose that these differences result primarily from relative changes in POC consumption in the upper water column.
Dymond and Collier (1996) described how POC rained out of the modern equatorial Pacific in relation to Ba. They found that lower levels of particulate POC rain away from the Equator corresponded to a much lower ratio of POC to Ba (∼30). In contrast, this ratio has a value of ∼150 near the Equator, where POC rain was high. The data suggest the relatively rapid formation of Ba in microenvironments within particulate rain, followed by a loss of POC relative to Ba. There is more complete consumption of POC in the surface ocean, where the POC flux is lower. The POC-to-Ba ratios found in sediments (Fig. 2) are much lower due to the high degradation of POC at the sea floor relative to Ba before burial (a reduction for POC vs. a reduction for Ba). However, the amount of Ba fixed in microenvironments depends on the Ba composition of seawater. In the modern oceans, Ba rain is significantly lower in the Atlantic than in the Pacific relative to POC, due to the lower levels of dissolved Ba in the Atlantic (Dymond and Collier, 1996).
If modern observations across the equatorial region are consistent with changes that might occur in a warm interval like the MCO, we expect there to be lower levels of POC in particulate rain relative to Ba. Conceivably, then, the POC rain might slow down, even though the Ba flux did not. This could happen if dissolved Ba is fixed into barite (BaSO4) relatively early in the rain of particulates, such that later degradation of POC only affects the POC : BaSO4 ratio as it leaves the surface waters (Fig. 3). However, the POC MAR resembles the opal MAR to a certain extent after the end of the MCO, indicating that the POC MAR has a profile similar to that of a different proxy for productivity when surface waters cool. Perhaps the presence of diatoms causes a more effective transport of particulate POC to the sea floor.
In time series for Site U1338, we find significant, non-random changes in the ratio of POC to Ba, associated with apparent changes in productivity (Fig. 3). Specifically, high POC : BaSO4 ratios are associated with the Late Miocene Biogenic Bloom and with a period around 12 Ma that also shows high levels of biogenic Si and CaCO3 deposition. These time series show that biogenic components have individual processes that lead from creation to burial, meaning care needs to be taken to quantitatively ascribe a certain level of primary productivity to the remains found in the sediments. Nevertheless, the lack of POC response during the MCO likely results from upper-water-column processes.
In earlier work, we found that warm Earth conditions during the Eocene were marked by very low levels of POC burial (Olivarez Lyle and Lyle, 2005, 2006). In this study, we show that warm Earth conditions during the early Miocene and the Miocene Climate Optimum were also characterized by low levels of POC burial compared to the later sedimentary record. These low levels are represented both in the weight percentage of POC and in POC MARs, as well as in the low ratios of POC relative to other, better-preserved biogenic components (such as BaSO4), despite the relatively high deposition of other paleoproductivity proxies (such as biogenic silica). We formed four hypotheses to explain the low POC levels during the MCO – lower productivity, higher degradation in the lower water column, higher degradation in the upper water column, and a change in the relationships between proxies – and rejected all of them except for higher degradation in the upper water column.
Our data suggest that POC is preferentially removed in the upper water column, indicating a “short circuit” in the biological pump under extreme global warmth once the ocean equilibrates. We observe that the average POC content of equatorial Pacific sediments from the MCO, the warmest Miocene interval, was about 0.04 %, which is 5 times lower than that of modern surface sediments (0.2 % to 0.3 %; Murray and Leinen, 1993). POC in modern surface sediments is roughly proportional to the rain of POC that reaches the sea floor (as measured by near-bottom sediment traps). During the MCO, it appears that ∼5 times less POC reached the sea floor, likely caused by higher metabolic degradation in surface waters.
While the proportion of particulate POC rain to POC burial is an oversimplification of early diagenesis in pelagic environments, it is an example of how the pelagic sedimentary environment responds to warm Earth conditions. Better diagenetic models accounting for low sedimentation rates and oxic conditions might improve our ability to hindcast particulate rain from the past. Clearly, however, there is a sedimentary response to these processes in the water column.
Code used for splicing is available at https://www.codd-home.net/ (CODD, 2023).
The data sets provided in the Supplement for this paper were submitted for archival on Pangaea (https://doi.pangaea.de/10.1594/PANGAEA.974057, Lyle and Olivarez Lyle, 2024).
The supplement related to this article is available online at: https://doi.org/10.5194/cp-20-2685-2024-supplement.
ML helped collect the cores from Sites U1337 and U1338. He organized the XRF analyses along the continuous sediment sections (splices) and secured funding for the discrete-calibration carbon samples. He had primary responsibility for writing the paper. AOL trained and supervised student analysts with regard to both bio-SiO2 and carbon analyses (CaCO3 and POC) and was responsible for the quality control of the resulting data. She also helped write and edit the paper.
The contact author has declared that neither of the authors has any competing interests.
Publisher's note: Copernicus Publications remains neutral with regard to jurisdictional claims made in the text, published maps, institutional affiliations, or any other geographical representation in this paper. While Copernicus Publications makes every effort to include appropriate place names, the final responsibility lies with the authors.
We thank the scientific party and crew of the R/V JOIDES Resolution who participated in IODP Expeditions 320 and 321 (conducted in 2009) for the collections made at and initial descriptions of Sites U1337 and U1338. We also thank the students and technical staff who ran the carbon analyses (Chris Piela, Bianca Romero, Julia Wilson, and Anna Stepanova). We also thank Joanna T. Lyle for reviewing the initial draft of this paper.
This research has been supported by the US National Science Foundation Directorate for Geosciences (grant no. 1656960).
This paper was edited by Yannick Donnadieu and reviewed by Baptiste Suchéras-Marx and one anonymous referee.
Behrenfeld, M. J., Boss, E. S., Siegel, D. A., and Shea, D. M.: Carbon-based ocean productivity and phytoplankton physiology from space, Global Biogeochem. Cy., 19, GB1006, https://doi.org/10.1029/2004GB002299, 2005.
Berelson, W. M.: Particle settling rates increase with depth in the ocean, Deep-Sea Res. Pt. II, 49, 237–251, 2001.
Berger, W. H.: Cenozoic sedimentation in the eastern tropical Pacific, Geol. Soc. Am. Bull., 84, 1941–1954, 1973.
Boscolo-Galazzo, F., Crichton, K. A., Barker, S., and Pearson, P. N.: Temperature dependency of metabolic rates in the upper ocean: A positive feedback to global climate change?, Global Planet. Change, 170, 201–212, https://doi.org/10.1016/j.gloplacha.2018.08.017, 2018.
Boscolo-Galazzo, F., Crichton, K. A., Ridgwell, A., Mawbey, E. M., Wade, B. S., and Pearson, P. N.: Temperature controls carbon cycling and biological evolution in the ocean twilight zone, Science, 371, 1148–1152, 2021.
Boyd, P. W.: Toward quantifying the response of the oceans biological pump to climate change, Front. Mar. Sci., 2, 77, https://doi.org/10.3389/fmars.2015.00077, 2015.
Bralower, T. J., Premoli Silva, I., Malone, M. J., Arthur, M. A., Averyt, K., Brassell, S. C., Bown, P. R., Channell, J. E. T., Clarke, L. J., Dutton, A., Eleson, J. W., Frank, T. D., Gylesjö, S., Hancock, H., Kana, H., Leckie, R. M., Marsaglia, K. M., McGuire, J., Petrizzo, M. R., Robinson, S., Röhl, U., Sager, W. W., Takeda, K., Thomas, D., Zachos, J.C., Williams, T., and Moe, K. T.: Proceedings of the Ocean Drilling Program, Initial Reports, Ocean Drilling Program, College Station, TX, https://doi.org/10.2973/odp.proc.ir.198.2002, 2002.
Broecker, W., Klas, M., Clark, E., Bonani, G., Ivy, S., and Wolfli, W.: The influence of CaCO3 dissolution on core top radiocarbon ages for deep-sea sediments, Paleoceanography, 6, 593–608, 1991.
Brown, J. H., Gillooly, J. F., Allen, A. P., Savage, V. M., and West, G. B.: Toward a metabolic theory of ecology, Ecology, 85, 1771–1789, 2004.
Chaisson, W. P. and Leckie, R. M.: High-Resolution Neogene Planktonic Foraminifer Biostratigraphy of Site 806, Ontong Java Plateau (Western Equatorial Pacific), in: Proceedings ODP, Scientific Results, leg 130, Ocean Drilling Program, College Station, TX, 137–178, https://doi.org/10.2973/odp.proc.sr.130.010.1993, 1993.
Chavez, F. P. and Barber, R. T.: An estimate of new production in the equatorial Pacific, Deep-Sea Res., 34, 1229–1243, 1987.
CODD: Code for Ocean Drilling Data, https://www.codd-home.net/ (last access: 8 August 2023), 2023.
Dehairs, F., Chesselet, R., and Jedwab, J.: Discrete suspended particles of barite and the barium cycle in the open ocean, Earth Planet. Sc. Lett., 49, 528–550, 1980.
Dickens, G. R. and Owen, R. M.: The latest Miocene-early Pliocene biogenic bloom: a revised Indian Ocean perspective, Mar. Geol., 161, 75–91, 1999.
Diester-Haass, L., Billups, K., and Emeis, K.-C.: In search of the late Miocene–early Pliocene “biogenic bloom” in the Atlantic Ocean (Ocean Drilling Program Sites 982, 925, and 1088), Paleoceanography, 20, PA4001, https://doi.org/10.1029/2005PA001139, 2005.
Drury, A. J., Westerhold, T., Frederichs, T., Tian, J., Wilkens, R., Channell, J. E. T., Evans, H., John, C. M., Lyle, M., and Röhl, U.: Late Miocene climate and time scale reconciliation: Accurate orbital calibration from a deep-sea perspective, Earth Planet. Sc. Lett., 475, 254–266, https://doi.org/10.1016/j.epsl.2017.07.038, 2017.
Drury, A. J., Lee, G. P., Gray, W. R., Lyle, M., Westerhold, T., Shevenell, A. E., and John, C. M.: Deciphering the state of the late Miocene to early Pliocene equatorial Pacific, Paleoceanogr. Paleoclimatol., 33, 246–263, https://doi.org/10.1002/2017PA003245, 2018.
Dugdale, R. C., Wilkerson, F. P., Barber, R. T., and Chavez, F. P.: Estimating new production in the equatorial Pacific Ocean at 150° W, J. Geophys. Res., 97, 681–687, 1992.
Dymond, J. and Collier, R.: Particulate barium fluxes and their relationships to biological productivity, Deep-Sea Res. Pt. II, 43, 1283–1308, 1996.
Dymond, J. and Lyle, M.: Particle fluxes in the ocean and implications for sources and preservation of ocean sediments, in: Material Fluxes on the Surface of the Earth, edited by: Hay, W. W., Andrews, J. T., Baker, V. R., Dymond, J., Kump, L. R., Lerman, A., Martin, W. R., Meybeck, M., Milliman, J. D., Rea, D. K., and Sayles, F. L., National Academy Press, Washington, D.C., 125–143, https://doi.org/10.17226/1992, 1994.
Dymond, J., Suess, E., and Lyle, M.: Barium in deep-sea sediment: A geochemical proxy for paleoproductivity, Paleoceanography, 7, 163–181, 1992.
Evans, H. F.: Magnetic Stratigraphy and Environmental Magnetism of Oceanic Sediments, Geology, PhD thesis, University of Florida, 204 pp., https://ufdc.ufl.edu/UFE0017566/00001 (last access: 6 December 2024), 2006.
Expedition 320/321 Scientists: Methods, in: Proc. IODP, 332/321, edited by: Pälike, H., Lyle, M., Nishi, H., Raffi, I., Gamage, K., Klaus, A., and the Expedition 320/321 Scientists, Integrated Ocean Drilling Program Management International, Inc., Tokyo, https://doi.org/10.2204/iodp.proc.320321.102.2010, 2010a.
Expedition 320/321 Scientists: Site U1337, in: Proc. IODP, 332/321, edited by: Pälike, H., Lyle, M., Nishi, H., Raffi, I., Gamage, K., Klaus, A., and the Expedition 320/321 Scientists, Integrated Ocean Drilling Program Management International, Inc., Tokyo, https://doi.org/10.2204/iodp.proc.320321.109.2010, 2010b.
Ganeshram, R. S., Francois, R., Commeau, J., and Brown-Leger, S. L.: An experimental investigation of barite formation in seawater, Geochim. Cosmochim. Ac., 67, 2599–2605, 2003.
Gastaldello, M. E., Agnini, C., Westerhold, T., Drury, A. J., Sutherland, R., Drake, M. K., Lam, A. R., Dickens, G. R., Dallanave, E., Burns, S., and Alegret, L.: The Late Miocene-Early Pliocene Biogenic Bloom: An Integrated Study in the Tasman Sea, Paleoceanogr. Paleoclimatol., 38, e2022PA004565, https://doi.org/10.1029/2022PA004565, 2023.
Guillermic, M., Misra, S., Eagle, R., and Tripati, A. K.: Atmospheric CO2 estimates for the Miocene to Pleistocene based on foraminiferal δ11B at Ocean Drilling Program Sites 806 and 807 in the Western Equatorial Pacific, Clim. Past, 18, 183–207, https://doi.org/10.5194/cp-18-183-2022, 2022.
Hedges, J. I. and Keil, R. G.: Sedimentary organic matter preservation: an assessment and speculative synthesis, Mar. Chem., 49, 81–115, 1995.
Holbourn, A., Kuhnt, W., Lyle, M., Schneider, L., Romero, O., and Andersen, N.: Middle Miocene climate cooling linked to intensification of eastern equatorial Pacific upwelling, Geology, 42, 19–22, https://doi.org/10.1130/G34890.1, 2014.
Holbourn, A., Kuhnt, W., Kochhann, K. G. D., Andersen, N., and Meier, K. J. S.: Global perturbation of the carbon cycle at the onset of the Miocene Climate Optimum, Geology, 43, 123–126, https://doi.org/10.1130/G36317.1, 2015.
Honjo, S., Manganini, S. J., and Poppe, L. J.: Sedimentation of Lithogenic Particles in the Deep Ocean, Mar. Geol., 50, 199–220, 1982.
Honjo, S., Dymond, J., Collier, R., and Manganini, S. J.: Export production of particles to the interior of the equatorial Pacific Ocean during the 1992 EqPac experiment, Deep-Sea Res., 42, 831–870, 1995.
John, E. H., Wilson, J. D., Pearson, P. N., and Ridgwell, A.: Temperature-dependent remineralization and carbon cycling in the warm Eocene oceans, Palaeogeogr. Palaeocl. Palaeoecol., 413, 158–166, https://doi.org/10.1016/j.palaeo.2014.05.019, 2014.
Kadko, D. and Heath, G. R.: Models of Depth-Dependent Bioturbation at Manop Site H in the Eastern Equatorial Pacific, J. Geophys. Res.-Oceans, 89, 6567–6570, 1984.
Karatsolis, B.-T., Lougheed, B. C., De Vleeschouwer, D., and Henderiks, J.: Abrupt conclusion of the late Miocene-early Pliocene biogenic bloom at 4.6–4.4 Ma, Nat. Commun., 13, 353, https://doi.org/10.1038/s41467-021-27784-6, 2022.
Kochann, K. G. D., Holbourn, A., Kuhnt, W., and Xu, J.: Eastern equatorial Pacific benthic foraminiferal distribution and deep water temperature changes during the early to middle Miocene, Mar. Micropaleontol., 133, 28–39, https://doi.org/10.1016/j.marmicro.2017.05.002, 2017.
Kroenke, L. W., Berger, W. H., Janecek, T. R., Backman, J., Bassinot, F., Corfield, R. M., Delaney, M. L., Hagen, R., Jansen, E., Krissek, L. A., Lange, C., Leckie, R. M., Linde, I. L., Lyle, M. W., Mahoney, J. J., Marsters, J. C., Mayer, L., Mosher, D. C., Musgrave, R., Prentice, M. L., Resig, J. M., Schmidt, H., Stax, R., Storey, M., Takahashi, K., Takayama, T., Tarduno, J. A., Wilkens, R. H., and Wu, G.: Proceedings of the Ocean Drilling Program, Initial Reports, Vol. 130, Ocean Drilling Program, College Station, TX, https://doi.org/10.2973/odp.proc.ir.130.1991, 1991.
Lear, C. H., Coxall, H. K., Foster, G. L., Lunt, D. J., Mawbey, E. M., Rosenthal, Y., Sosdian, S. M., Thomas, E., and Wilson, P. A.: Neogene ice volume and ocean temperatures: Insights from infaunal foraminiferal Mg/Ca paleothermometry, Paleoceanography, 30, 1437–1454, https://doi.org/10.1002/2015PA002833, 2015.
Li, Z., Zhang, Y. G., Torres, M., and Mills, B. J. W.: Neogene burial of organic carbon in the global ocean, Nature, 613, 90–95, https://doi.org/10.1038/s41586-022-05413-6, 2023.
Lyle, M.: Neogene carbonate burial in the Pacific Ocean, Paleoceanography, 18, 1059–1078, https://doi.org/10.1029/2002PA000777, 2003.
Lyle, M. and Baldauf, J.: Biogenic sediment regimes in the Neogene equatorial Pacific, IODP Site U1338: Burial, production, and diatom community, Palaeogeogr. Palaeocl. Palaeoecol., 433, 106–128, https://doi.org/10.1016/j.palaeo.2015.04.001, 2015.
Lyle, M., Olivarez Lyle, A., Gorgas, T., Holbourn, A., Westerhold, T., Hathorne, E. C., Kimoto, K., and Yamamoto, S.: Data report: raw and normalized elemental data along the U1338 splice from X-ray Fluorescence scanning, Proceedings of the Integrated Ocean Drilling Program, 320/321, US Implementing Organization Science Services, Texas A&M University, https://doi.org/10.2204/iodp.proc.320321.2010, 2012.
Lyle, M., Mix, A., Ravelo, C., Andreasen, D., Heusser, L., and Olivarez, A.: Millennial-scale CaCO3 and C-org events along the northern and central California margin: stratigraphy and origins, in: Proceedings of the Ocean Drilling Program, Scientific Results, edited by: Lyle, M., Koizumi, I., Moore Jr., T. C., and Richter, C., Ocean Drilling Program, College Station, TX, 163–182, 2000.
Lyle, M., Drury, A. J., Tian, J., Wilkens, R., and Westerhold, T.: Late Miocene to Holocene high-resolution eastern equatorial Pacific carbonate records: stratigraphy linked by dissolution and paleoproductivity, Clim. Past, 15, 1715–1739, https://doi.org/10.5194/cp-15-1715-2019, 2019.
Lyle, M. W. and Olivarez Lyle, A.: Data to reconstruct biogenic sedimentary component time series from the Miocene Pacific to study the biological pump [dataset bundled publication], PANGAEA [data set], https://doi.pangaea.de/10.1594/PANGAEA.974057, 2024.
Lyle, M. W., Olivarez Lyle, A., Backman, J., and Tripati, A.: Biogenic sedimentation in the Eocene equatorial Pacific: the stuttering greenhouse and Eocene carbonate compensation depth, in: Proceedings of the Ocean Drilling Program, Scientific Results, Leg 199, edited by: Lyle, M., Wilson, P., Janecek, T. R., and Firth, J., Ocean Drilling Program, College Station, TX, https://doi.org/10.2973/odp.proc.sr.199.219.2005, 2005.
Martin, J. H., Knauer, G. A., Karl, D. M., and Broenkow, W. W.: VERTEX: carbon cycling in the northeast Pacific, Deep-Sea Res., 34, 267–285, 1987.
Mayer, L. A.: Extraction of high-resolution carbonate data for palaeoclimate reconstruction, Nature, 352, 148–150, 1991.
Mayer, L. A., Theyer, F., Barron, J., Dunn, D. A., Handyside, T., Hills, S., Jarvis, I., Nigrini, C. A., Pisias, N., Pujos, A., Saito, T., Stout, P., Thomas, E., Weinrich, N., and Wilkens, R. H.: Initial Reports of the Deep Sea Drilling Program, Leg 85, US Government Printing Office, Washington, 1021 pp., https://doi.org/10.2973/dsdp.proc.85.1985, 1985.
Mayer, L. M.: Sedimentary organic matter preservation: an assessment and speculative synthesis-a comment, Mar. Chem., 49, 123–126, 1995.
McDonnell, A. M. P. and Buessler, K. O.: Variability in the average sinking velocity of marine particles, Limnol. Oceanogr., 55, 2085–2096, 2010.
Moore Jr., T. C., Backman, J., Raffi, I., Nigrini, C., Sanfilippo, A., Palike, H., and Lyle, M.: The Paleogene tropical Pacific: Clues to circulation, productivity, and plate motion, Paleoceanography, 19, PA3013, https://doi.org/10.1029/2003PA000998, 2004.
Moore Jr., T. C., Mitchell, N. C., Lyle, M., Backman, J., and Pälike, H.: Hydrothermal pits in the biogenic sediments of the equaotrial Pacific Ocean, Geochem. Geophy. Geosy., 8, Q03015, https://doi.org/10.1029/2006GC001501, 2007.
Muller, P. J. and Suess, E.: Productivity, sedimentation rate, and sedimentary organic matter in the oceans – I. Organic carbon preservation, Deep-Sea Res., 26A, 1347–1362, 1979.
Murray, R. W. and Leinen, M.: Chemical transport to the seafloor of the equatorial Pacific Ocean across a latitudinal transect at 135° W: tracking sedimentary major, trace, and rare earth element fluxes at the equator and the Intertropical Convergence Zone, Geochim. Cosmochim. Ac., 57, 4141–4163, 1993.
Olivarez Lyle, A. and Lyle, M.: Organic carbon and barium in Eocene sediments: Possible controls on nutrient recycling in the Eocene equatorial Pacific Ocean, in: Proceedings of the Ocean Drilling Program, Scientific Reports Volume 199, edited by: Wilson, P. A., Lyle, M., and Firth, J. V., Ocean Drilling Program, College Station, TX, 1–33, http://www-odp.tamu.edu/publications/199_IR/chap_106/chap_106.htm (last access: 8 December 2024), 2005.
Olivarez Lyle, A. and Lyle, M.: Organic carbon and barium in Eocene sediments: Is metabolism the biological feedback that maintains end-member climates?, Paleoceanography, 21, 1–13, https://doi.org/10.1029/2005PA001230, 2006.
Pälike, H., Lyle, M., Nishi, H., Raffi, I., Gamage, K., Klaus, A., and the Expedition 320/321 Scientists: Proceedings of the Integrated Ocean Drilling Program (IODP), v. 320/321, Integrated Ocean Drilling Program Management International, Inc., Tokyo, https://doi.org/10.2204/iodp.proc.320321.2010, 2010.
Piela, C., Lyle, M., Marcantonio, F., Baldauf, J., and Olivarez Lyle, A.: Biogenic sedimentation in the equatorial Pacific: Carbon cycling and paleoproduction, 12–24 Ma, Paleoceanography, 27, PA2204, https://doi.org/10.1029/2011PA002236, 2012.
Prahl, F. G., Muehlhausen, L. A., and Lyle, M.: An organic geochemical assessment of oceanographic conditions at MANOP Site C over the past 26,000 years, Paleoceanography, 4, 495–510, 1989.
Rea, D. K., Basov, L. A., Janecek, T. R., Palmer-Julson, A., Arnold, E., Barron, J. A., Beaufort, L., Bristow, J. F., deMenocal, P., Dubuisson, Q. J., Qiadenokov, A. Y., Hamilton, T., Ingram, L., Keigwin Jr., L. D., Keller, R. A., Kotilainen, A., Krissek, L. A., McKelvey, B., Morley, J. J., Okada, M., Olafsson, Q., Owen, R. M., Pak, D. Pedersen, T. F., Roberts, J. A., Rutledge, A. K., Shilov, V. V., Snoeckx, H., Stax, R., Tiedemann, R., and Weeks, R.: Proceedings of the Ocean Drilling Program, Initial Reports, 145, Ocean Drilling Program, College Station, TX, https://doi.org/10.2973/odp.proc.ir.145.1993, 1993.
Rousseaux, C. S. and Gregg, W. W.: Forecasting ocean chlorophyll in the equatorial Pacific, Front. Mar. Sci., 4, 236, https://doi.org/10.3389/fmars.2017.00236, 2017.
Ryan, W. B. F., Carbotte, S. M., Coplan, J., O'Hara, S., Melkonian, A., Arko, R., Weissel, R. A., Ferrini, V., Goodwillie, A., Nitsche, F., Bonczkowski, J., and Zemsky, R.: Global Multi-Resolution Topography (GMRT) synthesis data set, Geochem. Geophy. Geosy., 10, Q03014, https://doi.org/10.1029/2008GC002332, 2009.
Sosdian, S. M., Greenop, R., Hain, M. P., Foster, G. L., Pearson, P. N., and Lear, C. H.: Constraining the evolution of Neogene ocean carbonate chemistry using the boron isotope pH proxy, Earth Planet. Sc. Lett., 498, 362–376, https://doi.org/10.1016/j.epsl.2018.06.017, 2018.
Stax, R. and Stein, R.: 34. Long-Term Changes In The Accumulation Of Organic Carbon In Neogene Sediments, Ontong Java Plateau, Proceedings of the Ocean Drilling Program, Scientific Results, 130, 573–584, 1993.
Stephens, M. P., Kadko, D. C., Smith, C. R., and Latasa, M.: Chlorophyll-a and pheopigments as tracers of labile organic carbon at the central equatorial Pacific seafloor, Geochem. Cosmochim. Ac., 61, 4605–4619, 1997.
Suess, E.: Particulate organic carbon flux in the oceans – surface productivity and oxygen utilization, Nature, 288, 260–263, 1980.
Takayama, T.: Notes on Neogene calcareous nannofossil biostratigraphy of the Ontong Java Plateau and size variations of Reticulofenestra coccoliths, in: Proceedings ODP, Scientific Results, Leg 130, Ocean Drilling Program, College Station, TX, 179–230, https://doi.org/10.2973/odp.proc.sr.130.020.1993, 1993.
Tian, J., Ma, X., Zhou, J., Jiang, X., Lyle, M., Shackford, J. K., and Wilkens, R.: Paleoceanography of the east equatorial Pacific over the past 16 Myr and Pacific-Atlantic comparison: High resolution benthic foraminiferal δ18O and δ13C records at IODP Site U1337, Earth Planet. Sc. Lett., 499, 185–196, https://doi.org/10.1016/j.epsl.2018.07.025, 2018.
Westerhold, T., Marwan, N., Drury, A. J., Liebrand, D., Agnini, C., Anagnostou, E., Barnet, J. S. K., Bohaty, S. M., De Vleeschouwer, D., Florindo, F., Frederichs, T., Hodell, D. A., Holbourn, A. E., Kroon, D., Lauretano, V., Littler, K., Lourens, L. J., Lyle, M., Pälike, H., Röhl, U., Tian, J., Wilkens, R. H., Wilson, P. A., and Zachos, J. C.: An astronomically dated record of Earth's climate and its predictability over the last 66 million years, Science, 369, 1383–1387, 2020.
Wilson, J. K.: Early Miocene carbonate dissolution in the eastern equatorial Pacific, PhD thesis, Oceanography, Texas A and M University, 155 pp., https://hdl.handle.net/1969.1/153962 (last access: 8 December 2024), 2014.
Wyrtki, K.: An estimate of equatorial upwelling in the Pacific, J. Phys. Oceanogr., 11, 1205–1214, 1981.