the Creative Commons Attribution 4.0 License.
the Creative Commons Attribution 4.0 License.
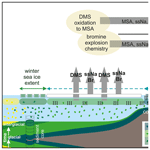
Antarctic sea ice over the past 130 000 years – Part 1: a review of what proxy records tell us
Xavier Crosta
Karen E. Kohfeld
Helen C. Bostock
Matthew Chadwick
Alice Du Vivier
Oliver Esper
Johan Etourneau
Jacob Jones
Amy Leventer
Juliane Müller
Rachael H. Rhodes
Claire S. Allen
Pooja Ghadi
Nele Lamping
Carina B. Lange
Kelly-Anne Lawler
David Lund
Alice Marzocchi
Katrin J. Meissner
Laurie Menviel
Abhilash Nair
Molly Patterson
Jennifer Pike
Joseph G. Prebble
Christina Riesselman
Henrik Sadatzki
Louise C. Sime
Sunil K. Shukla
Lena Thöle
Maria-Elena Vorrath
Wenshen Xiao
Jiao Yang
Antarctic sea ice plays a critical role in the Earth system, influencing energy, heat and freshwater fluxes, air–sea gas exchange, ice shelf dynamics, ocean circulation, nutrient cycling, marine productivity and global carbon cycling. However, accurate simulation of recent sea-ice changes remains challenging and, therefore, projecting future sea-ice changes and their influence on the global climate system is uncertain. Reconstructing past changes in sea-ice cover can provide additional insights into climate feedbacks within the Earth system at different timescales. This paper is the first of two review papers from the Cycles of Sea Ice Dynamics in the Earth system (C-SIDE) working group. In this first paper, we review marine- and ice core-based sea-ice proxies and reconstructions of sea-ice changes throughout the last glacial–interglacial cycle.
Antarctic sea-ice reconstructions rely mainly on diatom fossil assemblages and highly branched isoprenoid (HBI) alkenes in marine sediments, supported by chemical proxies in Antarctic ice cores. Most reconstructions for the Last Glacial Maximum (LGM) suggest that winter sea ice expanded all around Antarctica and covered almost twice its modern surface extent. In contrast, LGM summer sea ice expanded mainly in the regions off the Weddell and Ross seas. The difference between winter and summer sea ice during the LGM led to a larger seasonal cycle than today. More recent efforts have focused on reconstructing Antarctic sea ice during warm periods, such as the Holocene and the Last Interglacial (LIG), which may serve as an analogue for the future. Notwithstanding regional heterogeneities, existing reconstructions suggest that sea-ice cover increased from the warm mid-Holocene to the colder Late Holocene with pervasive decadal- to millennial-scale variability throughout the Holocene. Studies, supported by proxy modelling experiments, suggest that sea-ice cover was halved during the warmer LIG when global average temperatures were ∼2 ∘C above the pre-industrial (PI).
There are limited marine (14) and ice core (4) sea-ice proxy records covering the complete 130 000 year (130 ka) last glacial cycle. The glacial–interglacial pattern of sea-ice advance and retreat appears relatively similar in each basin of the Southern Ocean. Rapid retreat of sea ice occurred during Terminations II and I while the expansion of sea ice during the last glaciation appears more gradual especially in ice core data sets. Marine records suggest that the first prominent expansion occurred during Marine Isotope Stage (MIS) 4 and that sea ice reached maximum extent during MIS 2. We, however, note that additional sea-ice records and transient model simulations are required to better identify the underlying drivers and feedbacks of Antarctic sea-ice changes over the last 130 ka. This understanding is critical to improve future predictions.
- Article
(4202 KB) - Full-text XML
-
Supplement
(89 KB) - BibTeX
- EndNote
Sea ice is a vital component of the Southern Ocean (SO), exerting influence on water mass properties, ocean dynamics (Maksym, 2019) and ecosystem functioning (Massom and Stammerjohn, 2010) (Fig. 1). The formation of sea ice within large coastal polynyas around Antarctica results in brine rejection leading to the formation and sinking of dense shelf water (DSW). In some regions (Weddell Sea, Ross Sea, Adelie Land, Cape Darnley), this DSW contributes to the formation of Antarctic bottom water (AABW; Rintoul, 1998, 2018; Ohshima et al., 2013) which plays an important role in ventilating the bottom waters of the global ocean (Purkey et al., 2018). The melting of sea ice also adds buoyancy to waters that are upwelled in the SO, helping transform deep waters into mode and intermediate waters found in the Atlantic, Indian and Pacific basins (Abernathey et al., 2016; Pellichero et al., 2018). These SO intermediate waters represent the main source of nutrients for the thermocline and, ultimately, support low latitudes primary productivity (Sarmiento et al., 2004). Sea ice has been proposed as an important long-term modulator of global ocean circulation through its influence on surface buoyancy fluxes that control the interface between the shallow and deep SO overturning cells (Ferrari et al., 2014) and the overturning rate of the deep ocean (Galbraith and Skinner, 2020).
Sea-ice cover also influences atmospheric energy fluxes by reducing the solar heating (ice–albedo effect) of the ocean (Hall, 2004), air–sea fluxes of sensible and latent heat and by reducing the vertical ocean mixing (surface stratification effect) when sea ice melts (Goosse and Zunz, 2014; Lecomte et al., 2017; Maksym, 2019). Landfast ice (sea ice that is attached to icebergs or land) has also been shown to dampen the mechanical impact of ocean swell onto ice shelves that are flowing out of the Antarctic ice sheet, therefore, increasing the ice shelves' stability and preventing them from calving (Greene et al., 2018; Massom et al., 2018).
Sea ice has a strong influence on nutrient and carbon cycling along with marine ecosystem functioning throughout the SO. Sea-ice formation in autumn and winter results in the sinking of CO2-enriched brine while the sea-ice cover prevents the exchange of CO2 between the surface waters and the atmosphere (Arrigo and van Dijken, 2007; Rysgaard et al., 2011). In spring and summer, sea-ice melt forms a low-density lid enriched in micro- and macro-nutrients at the ocean surface (Lannuzel et al., 2010) supporting biological productivity that acts as a carbon sink (Vancoppenolle et al., 2013; Takao et al., 2020). Another area of high biological productivity are in polynyas where open water surrounded by sea ice often supports dense algal blooms (Arrigo and van Dijken, 2003; Arrigo et al., 2015; DeJong and Dunbar, 2017) that subsequently die and sink to the bottom, transferring large amount of organic carbon to the seafloor (DeJong and Dunbar, 2017). In these sea-ice environments, diatoms and Phaeocystis represent the main primary producers (Wright and van den Enden, 2000; Wright et al., 2010) and vectors of carbon to the sea floor (Nissen and Vogt, 2021) with diatoms generally being dominant in more stratified surface waters (Di Tullio et al., 2000; Arrigo et al., 2010). Sea-ice presence can also have direct or indirect impacts on other components of the Antarctic marine ecosystem (Massom and Stammerjohn, 2010). Phytoplankton within sea-ice melt or coastal polynyas provides the primary food source for zooplankton and the cascading food chain (Eicken, 1992; Loeb et al., 1997; Norkko et al., 2007; Ainley et al., 2017; Labrousse et al., 2018; Wing et al., 2018; Rossi et al., 2019). Sea ice also provides a direct substrate for algae, and an important resting and breeding platform for large predators such as penguins and seals (Fraser et al., 1989; Ancel et al., 1992; Labrousse et al., 2017). Thus, Antarctic sea ice plays an important physical, biogeochemical and ecological role that is observed around the Antarctic margin, the SO and further afield.
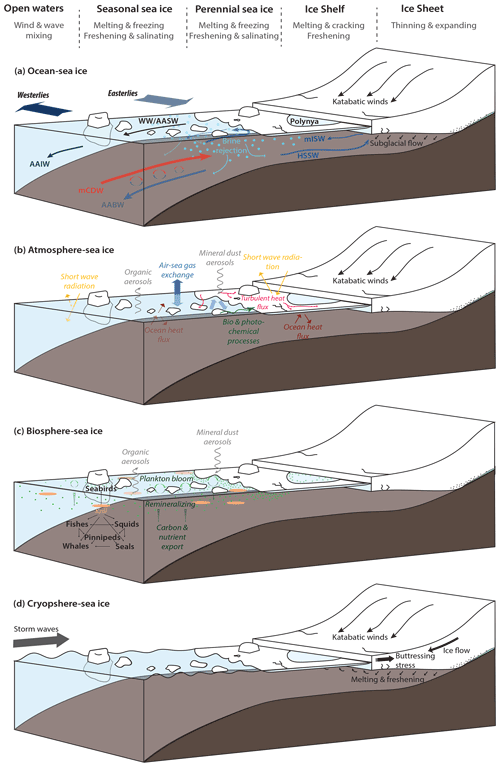
Figure 1Major feedbacks and interactions between Antarctic sea ice and the ocean, biosphere, atmosphere and cryosphere. WW: winter water, AASW: Antarctic surface water; AAIW: Antarctic intermediate water; mCDW: modified circumpolar water; AABW: Antarctic bottom water; HWWS: high salinity shelf water; mISW: modified ice shelf water.
After decades of expansion (Hobbs et al., 2016; Comiso et al., 2017), Antarctic sea ice has been declining since 2014 with satellite images showing Antarctic summer and winter sea ice (SSI and WSI, respectively) at a minimum compared to the average for the 1981–2010 period (Parkinson, 2019; Parkinson and DiGirolamo, 2021). The causes of this expansion and subsequent decline are not yet fully understood but may be related to complementary processes such as deepening of the ozone hole (Ferreira et al., 2015), freshening of surface waters due to ice-shelf melt (Bintanja et al., 2013, 2015; Rye et al., 2020) or changes in atmospheric circulation, wind stress and thermodynamic processes linked to the Southern Annular Mode (SAM) and El Niño–Southern Oscillation (ENSO) (Hall and Visbeck, 2002; Holland and Kwok, 2012; Matear et al., 2015; Kwok et al., 2016; Turner et al., 2016; Kusahara et al., 2019; Maksym, 2019; Yang et al., 2021; Fogt et al., 2022). Climate models that were part of the Third, Fifth and Sixth Coupled Model Intercomparison Projects (CMIP3, CMIP5 and CMIP6, respectively), used by the United Nations Intergovernmental Panel on Climate Change (IPCC), have predicted that the WSI extent is expected to decline between 24 % and 34 % by 2100 (Arzel et al., 2006; Bracegirdle et al., 2008; IPCC, 2013; Roach et al., 2020). The greatest declines are expected in the Amundsen, Bellingshausen and Weddell seas. The projected changes in sea ice over the coming century are expected to have implications for changes in ocean (Swingedouw et al., 2008) and atmospheric circulation patterns (England et al., 2020), heat transport, marine productivity (Arrigo et al., 2008) and nutrient and carbon cycling (Pant et al., 2018; Vernet et al., 2019). However, models do not capture the overall observed sea-ice trends or regional variability over the historical period (Maksym et al., 2012; Turner et al., 2013; Zunz et al., 2013; Maksym, 2019) and there remains uncertainty about sea ice parametrization (Blockley et al., 2020) and the role of mesoscale eddies in sea-ice area trends (Rackow et al., 2022). Thus, projections of future Antarctic sea-ice extent and the associated climate implications are highly uncertain.
Quantifying past changes in sea ice and its influence on the Earth system is one approach for better understanding the short- and long-term feedbacks of sea ice in different climatic contexts, and to provide the data necessary to test our sea-ice modelling capabilities. Our understanding of past sea-ice dynamics over the Pleistocene is based on a limited number of sediment and ice core records. The C-SIDE working group (Chadwick et al., 2019; Rhodes et al., 2019) recently compiled an inventory of published marine records that have the potential to provide evidence of changes in sea ice during the past 130 000 years. In the present paper, we review how past changes in sea ice are reconstructed from marine and ice core proxies, and we summarise sites with existing records and present reconstructions for key periods of time such as the Last Glacial Maximum, Holocene and warmer-than-PI past interglacial periods. Section 2 describes our current understanding of how sea ice is changing and some of the challenges faced by models in reproducing these changes. Section 3 describes the proxies used to reconstruct past sea-ice conditions while Sect. 4 communicates what we currently know (and do not know) about past sea-ice changes. Section 4 mainly focuses on marine records that allow the reconstruction of the WSI and SSI extent during key periods of time. Finally, Sect. 5 gives some future directions for Antarctic sea-ice research.
2.1 Formation and decay processes
Sea ice forms from the freezing of ocean water. The large decrease in solar energy at high Southern latitudes during austral autumn–winter (Van Den Broeke et al., 2005) cools the atmosphere which favours the dissipation of ocean sensible heat to the atmosphere, hence, cooling the surface water layer (Gordon, 1981; Tamsitt et al., 2017). Initial ice crystals form when ocean water reaches a salinity-dependent freezing temperature (−1.9 ∘C for sea water with a salinity of ∼34 psu) (Petrich and Eicken, 2017). Abundant solid impurities present in the ocean accelerate ice crystal nucleation with individual crystal growing up to few millimetres in diameter but less than a millimetre in thickness (Weeks and Ackley, 1982). Further freezing, accretion and consolidation by winds, ocean currents, waves and swell subsequently produce centimetre-large aggregates (frazil ice) which then form decimetre-large floes/pans in the presence of surface ocean waves (pancake ice). Ultimately, pans are agglomerated in a consolidated sheet that thickens via congelation at the ocean–ice interface and snow accumulation and subsequent flooding at the atmosphere–ice interface (Sturm and Massom, 2017). This consolidated pack-ice `lid' drastically reduces heat dissipation to the atmosphere which provides a negative feedback on sea-ice vertical growth and limits its thickness to ∼1 m (Worby et al., 2008; Petrich and Eicken, 2017). However, thicker sea ice can be found in coastal areas around Antarctica due to dynamic convergence and accretion of platelet ice below the initial sea-ice sheet (Hoppman et al., 2020). Platelet ice are lamellar plates 2–15 cm wide and 1–2 mm thick formed by the supercooling of ice shelf water at depth which, due to positive buoyancy, floats up to the surface below the congealed sea-ice layer (Dieckmann et al., 1986; Langhorne et al., 2015). Large polynyas can be present between the thicker, sometimes multi-year, coastal fast sea ice and the thinner pack ice, and serve as “sea-ice factories”. Most of these polynyas are latent heat polynyas (formed by winds) where new sea ice is continuously formed and transported northward by ocean currents and katabatic winds (Massom et al., 1998).
At present, sea ice reaches a peak extent of km2 in September (Cavalieri et al., 2003; Cavalieri and Parkinson, 2008) and covers a large part of the SO. The WSI limit reaches as far north as ∼55∘ S in the Atlantic and western Indian sectors, ∼60∘ S in the central Indian sector and 62–65∘ S in the eastern Indian and Pacific sectors (Hobbs et al., 2016). The maximum extent is a balance between sea-ice gain from surface water freezing, equatorward transport and sea-ice loss at the margin by ocean and atmosphere-induced melting and mechanical break-up (Ackley, 1980; Comiso, 2003). Greater sea-ice extent in the Atlantic and western Pacific sectors is due to intense northward transport by the Weddell and Ross oceanic gyres (Olbers et al., 1992; Comiso, 2003; Nicholls et al., 2009).
Sea-ice decay starts in austral spring when solar energy at Southern high latitudes increases in addition to ocean heat due to direct intrusion of warm waters from lower latitudes (Comiso, 2003). The atmosphere-to-ocean heat flux and the deep-to-surface ocean heat flux were initially thought to play equal roles in sea-ice decay (Gordon, 1981). However, recent models suggest that upwelling of warm water below the ice pack promotes sea-ice thinning through bottom melt which eventually drives sea-ice spring–summer retreat (Singh et al., 2020). Mechanical breakup at the ice margin and absorption of solar radiation by the ice-free surface ocean in increasingly large leads within the sea ice provide additional positive feedbacks to sea-ice melting and accelerate its retreat (Ackley, 1980; Gordon, 1981; Holland, 2014). Mean summer sea-ice extent amounts to km2 in February–March and is essentially restricted to the Weddell, Ross and Amundsen seas' embayments (Cavalieri et al., 2003; Cavalieri and Parkinson, 2008). Overall, the Antarctic sea-ice seasonal cycle is asymmetric with a faster decay in spring than formation in autumn.
2.2 Recent sea-ice changes
The satellite era has allowed a precise assessment of Antarctic seasonal sea-ice cover since 1979. Over this period, high spatial variability has been observed in seasonal-to-interannual trends in maximum and minimum sea-ice extents, concentrations and thickness (Parkinson and Cavalieri, 2012; Yuan et al., 2017; Wang and Wu, 2021). Overall, Antarctic sea-ice extent increased slightly with a significant trend between 1979 and 2014 (Simmonds, 2015; Parkinson, 2019). The trend was significant for all seasons but more pronounced for the fall–winter period (Cavalieri and Parkinson, 2008). The slight increase in total extent was the result of opposing trends in different regions of Antarctica with a large decrease in sea-ice extent in the Amundsen and Bellinghausen seas offset by a large increase in the western Ross Sea (Zwally et al., 2002; Holland and Kwok, 2012; Fan et al., 2014; Jena et al., 2018; Parkinson, 2019). This regional and inter-annual variability has mainly been attributed to the atmospheric climate modes prevailing over the SO, such as the Antarctic Circumpolar Wave (White and Peterson, 1996; Raphael, 2007; Fogt et al., 2022) and the SAM (Kwok and Comiso, 2002; Simpkins et al., 2012; Kohyama and Hartmann, 2015), along with teleconnections to low latitude climate modes such as ENSO (Liu et al., 2002; Yuan, 2004; Hobbs and Raphael, 2010; Deb et al., 2014; Ciasto et al., 2015; Kohyama and Hartmann, 2015; Meehl et al., 2016). After years of increasing extent, there was an exceptional decline in Antarctic sea-ice extent in 2016 (Parkinson et al., 2019), especially in the Weddell and Ross seas (Hao et al., 2021). The 2016 minimum has been attributed to a combination of factors including decades of ocean warming, weakening of Southern Hemisphere westerly winds and increased advection of warmer air masses from low latitudes (Doddridge and Marshall, 2017; Nicolas et al., 2017; Stuecker et al., 2017; Turner et al., 2017; Alkama et al., 2020; Eayrs et al., 2021; Sabu et al., 2021). A small rebound in sea-ice extent has been observed in 2020 (Parkinson and DiGirolamo, 2021).
CMIP models simulate a large range of responses in Antarctic sea-ice extent and remain unable to capture some of the recently observed sea-ice trends. Most CMIP models simulate a decrease in WSI and SSI over the satellite period (Landrum et al., 2012; Turner et al., 2013; Gagné et al., 2015; Roach et al., 2020) and underestimate ice thickness (Shu et al., 2015). This mismatch may be the result of several factors. For example, the simulated sea-ice characteristics in CMIP models correlate closely with the simulated wind regimes. Some models do not adequately simulate the recent observed intensification and southward migration of the Southern Hemisphere westerly winds (SHWW) (Purich et al., 2016) and the associated poleward advection of warm waters into the Permanently Open Ocean Zone (POOZ) (Delworth and Zeng, 2008; Sigmond and Fyfe, 2010). Although the sea-ice models are increasingly sophisticated (Vancoppenolle et al., 2009; Hunke et al., 2015), the inaccurate representation of polynyas (Mohrmann et al., 2021) and SHWW location can impact the ice dynamics in models, including sea-ice melt through evaporation and sublimation (Andreas and Ackley, 1982) and sea-ice formation by floe accretion and sea-ice transport (Wadhams et al., 1987). Inclusion of more realistic floe size distribution may improve representation of the effects of wind-driven waves on ice growth and break-up (Roach et al., 2019). Some important processes may also be missing in the models. Snow ice formation, which occurs as snow on sea ice is submerged or washed with ocean waves, is particularly important in the SO and likely not well-represented (Jeffries et al., 2001; Massom et al., 2001). Additionally, the impact of mesoscale eddies on sea-ice accretion and transport is not resolved in the relatively coarse resolution CMIP class models (Hewitt et al., 2020; S. Li et al., 2021), an important shortcoming given that more recent high resolution climate models simulate a more realistic trend in sea-ice loss over the instrumental period (Rackow et al., 2022). Finally, the diversity in simulated sea-ice conditions may arise from different model responses to climatic modes of variability and atmosphere–ocean–ice interactions at different timescales (Holland et al., 2017; Kusahara et al., 2019). Given the shortcoming mentioned above, models cannot currently robustly assess whether the observed changes in sea ice over the last decades are part of natural climate variability or a response to anthropogenic forcing (Polvani and Smith, 2013; Hobbs et al., 2014; Jones et al., 2016; Eayrs et al., 2021).
Our current understanding of the drivers of decadal to multi-decadal variability in Antarctic sea ice is limited by the brevity of the satellite record (1979–present) and sparse distribution of observations on longer timescales. A longer term understanding of Antarctic sea-ice extent, offered by palaeoclimate data, is crucial to document the natural variability of sea ice, its drivers and feedbacks on the other climatic component from decadal to glacial–interglacial timescales. These data are also pivotal for identifying the pace of sea-ice climate responses under different mean conditions (such as time periods that were warmer than present) and during climate transitions. Past sea-ice changes are reconstructed using proxies such as fossil diatom assemblages and specific biomarkers archived in marine sediments, and geochemical tracers in polar ice cores (Fig. 2). Advantages and limitations of each proxy and approach are summarised in Table S1.
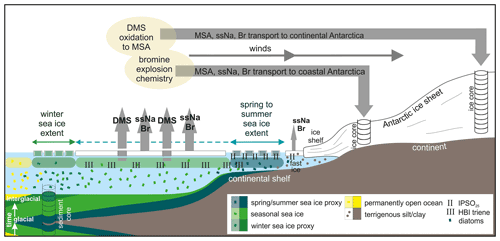
Figure 2Relationships between sea ice and sea-ice proxies found in marine sediment and ice core records. HBI: highly branched isoprenoids; IPSO25: ice proxy for the Southern Ocean with 25 carbon atoms; DMS: dimethyl sulfide; MSA: methane sulfonic acid; ssNA: sea salt sodium; Br: bromine compounds.
3.1 Diatoms
Diatoms preserved in marine sediments have been used for over 40 years as a way of reconstructing past changes in Antarctic sea ice and sea surface temperatures (SST) (Armand et al., 2017). Diatoms are phototrophic algae living in the euphotic zone and thus represent environmental conditions of the upper water column. In the SO, diatom assemblages are useful tools for reconstructing past SST because diatoms are widely distributed and their biogeographic distribution patterns are closely related to surface water temperature (e.g. Zielinski and Gersonde, 1997; Zielinski et al., 1998; Armand et al., 2005; Crosta et al., 2005; Romero et al., 2005; Esper et al., 2010). Furthermore, the abundance patterns of diatoms, specific diatom species or diatom assemblages are powerful tools for reconstructing Antarctic sea-ice conditions because specific species are found thriving at the sea-ice edge or attached to the sea ice (Armand et al., 2017).
Early works (e.g. Hays et al., 1976; DeFelice, 1979a; Cooke and Hays, 1982; Burckle, 1983) used surface sediment lithology for mapping Antarctic sea-ice extent with diatom-rich oozes found north of the modern WSI edge and diatomaceous muds and pelagic clays under sea-ice covered water (DeFelice, 1979b; Burckle et al., 1982). Using these modern sediment lithofacies as a guide, past large-scale sea-ice changes (e.g. glacial–interglacial cycles) were identified in the sediment record (DeFelice, 1979a). The lithological approach was further developed by relating sedimentary biogenic opal content to the WSI extent under the assumption that the majority of sediment-forming diatoms live in open ocean conditions between the WSI edge and the Subantarctic Front (Burckle and Cirilli, 1987; Burckle and Mortlock, 1998). Modern sediments show a strong negative relationship between their biogenic opal content and the overlying yearly sea-ice concentration which can be extended back through the sediment record to reconstruct past sea-ice concentrations (Burckle and Mortlock, 1998). Reconstructed sea-ice concentrations produced in this way have a sizeable error (±30 %, Burckle and Mortlock, 1998) however, and low biogenic opal content could also be related to temperature and/or nutrient constraints on diatom productivity (Neori and Holm-Hansen, 1982; Chase et al., 2015), dissolution and/or dilution of the biogenic opal (Zielinski et al., 1998) or reworking of sediments by bottom currents.
The diatom species assemblage preserved in marine sediments provides a more robust and precise method for reconstructing past sea-ice extent and duration. While diatoms make up almost two-thirds of the biota in modern sea ice (Garrison et al., 1986), many of these species that thrive on sea ice are too weakly silicified to be preserved in the underlying sediment (Leventer, 1998). However, several semi-quantitative approaches using more silicified sea-ice related species have been proposed. Burckle (1984) suggested the abundance pattern of the diatom species Eucampia antarctica as a sea-ice indicator while Kaczmarska et al. (1993) defined an Eucampia index calculated as the ratio of terminal to intercalary valves to trace the winter sea-ice field. Whitehead et al. (2005) improved the latter approach by calibrating the index with satellite-derived sea-ice data. Leventer (1992) and Leventer et al. (1993) suggested the relative abundance of Chaetoceros and the ratio of Chaetoceros resting spores to vegetative cells to be a potential tool for sea-ice reconstruction. Pike et al. (2009) proposed that the relationship between resting spores of Porosira glacialis and Thalassiosira antarctica has a potential as a semi-quantitative sea-ice proxy with ratio values >0.1 indicative of sea-ice concentration above 80 % and sea-ice duration greater than 7.5 months per year. However, these proxies have not been widely used probably because of the lack of large-scale modern validation.
Gersonde and Zielinski (2000) used information from sediments traps on the timing and magnitude of diatom fluxes from the ocean surface to the seafloor, along with considering diatom preservation and biogenic sediment accumulation, to assess whether diatoms can be used to estimate past WSI and SSI extent. They showed that the combined relative abundances of the more robustly silicified species Fragilariopsis curta and Fragilariopsis cylindrus (FCC), two species thriving at or below sea-ice margin (Burckle et al., 1987; von Quillfeldt, 2004) (Fig. 2), could be considered a qualitative tool to locate the edge of the mean WSI extent (Gersonde et al., 2003). Relative abundances of >3 % correspond roughly to an average WSI extent with mean sea-ice concentrations of 50 %–80 % (Gersonde et al., 2005). FCC values between 1 % and 3 % are considered to mark the maximum WSI extent (mean September sea-ice concentrations <20 %) (Gersonde et al., 2003, 2005). It should be noted, however, that the FCC proxy cannot be applied to sediments containing poorly preserved diatom assemblages, i.e. where selective dissolution could alter the relative abundances. Furthermore, because the FCC signal is produced during the spring/summer melt-back of the WSI, it is unrelated to the seasonal duration of the sea ice. Reconstruction of the SSI extent is more challenging. This is because regions covered by sea ice for long periods of the year experience low opal export, poor preservation and high opal dissolution which obscure the fossil signal of the SSI edge (Gersonde and Zielinski, 2000). However, the cold-water species (summer sea surface temperature, SSST, ∘C) Fragilariopsis obliquecostata is associated with year-long sea ice and is robustly silicified enough to be preserved in the sediment record (Zielinski and Gersonde, 1997; Gersonde and Zielinski, 2000). This allows the F. obliquecostata relative abundance threshold of >3 % to be used as an indicator of the mean February SSI extent (Gersonde and Zielinski, 2000). The above mentioned limitations suggest that the FCC and F. obliquecostata proxies are generally considered as threshold responses that reflect presence/absence of overlying sea ice rather than sea-ice duration (e.g. month per year). However, it should be noted that the relative abundances of both FCC and F. obliquecostata in modern sediments increase southward with increasing sea-ice concentration and duration (Zielinski and Gersonde, 1997; Armand et al., 2005; Esper et al., 2010) because these species are adapted to very short growing seasons. Therefore, high relative abundances of these species can still provide valuable qualitative information on sea-ice duration.
Multiple studies have used inverse statistical models to reconstruct quantitative estimates of past sea-ice concentration and duration using diatom assemblage-based transfer functions (e.g. Crosta et al., 1998; Whitehead and McMinn, 2002; Esper and Gersonde, 2014; Ferry et al., 2015b), with the most popular models being the Imbrie and Kipp Method (IKM; Imbrie and Kipp, 1971) and the Modern Analog Technique (MAT; Hutson, 1980). Crosta et al. (1998, 2004) made significant progress using the Modern Analog Technique to reconstruct yearly sea-ice duration in terms of months per year coverage while Esper and Gersonde (2014) developed MAT and IKM approaches to generate quantitative winter (September) sea-ice concentrations. These approaches generally make use of a large proportion of the diatom assemblage by incorporating around 30 diatom species that generally present a positive or negative relationship to sea-ice duration or sea-ice concentration. A different statistical approach is presented by Ferry et al. (2015b) who applied a generalised additive model (GAM) that only uses diatoms with statistically significant associations and ecologically-based links with sea ice. It is important to note that GAM and MAT approaches, despite being based on different statistical approaches, gave similar reconstructions of sea ice over the past 200 ka in a marine sediment core (SO136-111) from the southwest Pacific sector of the SO (Ferry et al., 2015a). The MAT and IKM methods also provided comparable reconstructions of WSI concentration in two cores (PS58/271-1 and PS1768-8) from the Pacific and Atlantic sectors of the SO (Esper and Gersonde, 2014). These results give strong confidence in the diatom transfer function tool to quantitatively estimate past sea-ice changes. These methods generally yield calibration errors on the modern model of about 1 month per year for sea-ice duration (Crosta et al., 1998, 2004) and 10 % for WSI concentration (Esper and Gersonde, 2014).
Like other proxy methods, diatom-based reconstructions of sea ice are dependent on various assumptions and face certain limitations (Chandler and Langebroek, 2021a). First, all reconstruction methods rely on the assumption that specific diatom species are adapted to use sea ice as a habitat (Thomas and Dieckmann, 2002; Bayer-Giraldi et al., 2010; van Leeuwe et al., 2018) and that this association has not changed through time, i.e. the principle of actualism. Another assumption is that fossil assemblages still track surface conditions despite the important and selective loss of diatoms during settling and burial. Indeed, around 1 %–5 % of the diatoms produced in the surface ocean reach the sediment (Ragueneau et al., 2000) and lightly silicified diatoms are preferentially lost in the first hundreds of metres of the water column (Lafond et al., 2020) and at the water–sediment interface (Rigual-Hernandez et al., 2016). Selective dissolution of less robustly silicified diatom valves results in an overall dominance of a few robust species in the surface and the down-core sediment record (Zielinski et al., 1998; Esper and Gersonde, 2014). Despite selective dissolution, many studies have shown that the distribution of the main diatom species in the phytoplankton (Hasle, 1969) is preserved in the surface sediment (Zielinski et al., 1998; Armand et al., 2005; Esper et al., 2010) and that winter sea-ice concentration and duration can be robustly reconstructed through diatom-based transfer functions (Crosta et al., 1998; Esper and Gersonde, 2014). Notwithstanding, poor preservation may complicate the reconstruction of past sea-ice changes in the region south of the modern WSI edge especially in cores located beneath year-long SSI. In extreme cases, very poor preservation may lead to non-analogue condition where fossil assemblages are composed of taxa in numbers that exceed their abundance in the surface sediment reference data set or are dominated by extinct diatom species. In this extreme case, transfer functions produce non-trustable, generally too warm estimates (IKM, GAM approaches) or are even unable to provide quantitative estimates (MAT). Regions close to the Antarctic continent require other proxies to complement the diatom record.
3.2 Highly branched isoprenoids (HBIs)
A relatively new tool for past sea-ice reconstructions are highly branched isoprenoid (HBI) alkenes that are produced by certain diatoms and are generally well preserved in marine sediments (Massé et al., 2011; Belt, 2018). A specific C25-HBI di-unsaturated alkene (or diene), more recently termed IPSO25 standing for ice proxy for the Southern Ocean with 25 carbon atoms, has been shown to be produced by the sympagic diatom Berkeleya adeliensis (Belt et al., 2016) which is a common constituent in platelet, bottom and landfast ice in Antarctic coastal environments (Riaux-Gobin and Poulin, 2004; Riaux-Gobin et al., 2013; Belt et al., 2016) (Fig. 2). Very few studies exist on the modern distribution of HBIs and the first analyses were done directly in sediment cores from the Antarctic continental shelf which had existing diatom counts (Barbara et al., 2010; Denis et al., 2010). However, the few studies performed so far have shown that the concentration of the HBI diene in water and surface sediment samples increases towards the Antarctic coast where heavy sea ice persists in spring–summer (Massé et al., 2011; Smik et al., 2016; Belt, 2018; Vorrath et al., 2019; Lamping et al., 2021). These studies also established the presence of tri-unsaturated C25-HBI alkenes (or trienes) with the HBI z-triene mostly biosynthesised by open ocean diatoms such as those belonging to the genus Rhizosolenia (Belt et al., 2017). An increased abundance of the HBI triene in surface waters and underlying sediments is associated with enhanced phytoplankton production near the marginal ice zone (Collins et al., 2013; Smik et al., 2016). Thus, paired records of IPSO25 and the HBI triene, and especially the ratio of the two biomarkers, reflect the relative contributions of sea-ice algae and open-water algae to phytoplankton productivity and, therefore, allow for improved reconstructions of seasonal sea-ice conditions/ice margin position (Barbara et al., 2010, 2016; Denis et al., 2010; Massé et al., 2011; Etourneau et al., 2013; Weber et al., 2022). Organic compounds such as HBIs may undergo some degradation, especially the triene, through abiotic and bacterial degradation in the water column and during early diagenesis in the sediments (Sinninghe Damsté et al., 2007; Massé et al., 2011; Rontani et al., 2014; 2019) or in laboratory repositories if storage conditions are not optimised (Sinninghe Damsté et al., 2007; Cabedo-Sanz et al., 2016). Differential effects of degradation on the HBI diene and triene might bias the diene/triene ratio observed in sediment records (Rontani et al., 2019). Therefore, caution is advised before analysing and interpreting HBI data with respect to past sea-ice conditions. HBIs have been measured back to 60 ka BP in deep-sea cores from the Scotia Sea with down-core variations showing good agreement with the diatom assemblage sea-ice proxies (Collins et al., 2013). This analysis indicates that the HBIs, whether locally produced or advected, can remain very well preserved in marine sediments for long periods of time.
Because of the lack of calibration studies, IPSO25 and diene/triene proxies have so far only provided qualitative information with higher values suggesting the presence of heavy sea-ice conditions. Fortunately, a semi-quantitative approach for HBI-based sea-ice reconstructions in the SO – the PIPSO25 index – has recently been proposed by Vorrath et al. (2019) and further developed by Vorrath et al. (2020) and Lamping et al. (2021). The PIPSO25 index is determined as the ratio of IPSO25 to the sum of IPSO25 and a phytoplankton biomarker (HBI triene or phytoplankton sterols; Vorrath et al., 2019). PIPSO25 values in surface sediments off the Amundsen Sea, the northern Antarctic Peninsula and from the southern Weddell Sea appear to show a positive correlation with sea-ice concentration derived from satellite observations and diatom transfer functions (Vorrath et al., 2019, 2020; Lamping et al., 2021). Importantly, the consideration of a phytoplankton biomarker alongside IPSO25 helps to avoid the misinterpretation of the absence of the sea-ice biomarker in a sediment sample which may result from ice-free conditions or perennial sea ice and/or ice shelf cover, both conditions inhibiting any ice algae productivity (Lamping et al., 2021). Therefore, while the PIPSO25 index seems a promising tool, further investigations of other environmental parameters, such as nutrient and light availability that affect bottom-ice algal growth (Kennedy et al., 2020), and of ice-shelf processes such as the formation and accretion of platelet ice that offer habitat to IPSO25-synthesising diatoms, are required to obtain more information on the production and fate of IPSO25 and better constrain its applicability as an Antarctic sea-ice proxy (Lamping et al., 2021). Further attempts to calibrate the PIPSO25 index against observational (i.e. satellite) sea-ice data also require higher circum-Antarctic spatial coverage of HBI analyses conducted on well-dated, ideally by 210Pb and 14C, surface sediments.
3.3 Other approaches: foraminifera, radiolaria, dinoflagellates
A new approach using oxygen isotope ratios (δ18O) of planktonic and benthic foraminifera was recently proposed to reconstruct WSI extent (Lund et al., 2021). The method relies on the fact that winter sea-ice formation creates a cold, surface mixed layer that persists in sub-surface layers during the spring and summer months. In the SO, this cold “winter water” rests above relatively warmer, deep water and creates an inverted temperature profile that is reflected in estimates of the equilibrium δ18O of calcite. Spatial mapping shows that winter water isotherms parallel the modern WSI edge throughout the SO. Additionally, published foraminiferal data from the Atlantic sector yield an estimate of WSI edge consistent with modern observations (Lund et al., 2021). The δ18O method is promising because it is grounded in hydrographic conditions associated with sea-ice formation and it takes advantage of foraminiferal δ18O measurements already used for stratigraphic purposes. However, the method is based on the assumption that winter water consistently tracks WSI extent and that the planktonic foraminifera (Neogloboquadrina pachyderma) primarily calcifies in winter water. Furthermore, the approach is necessarily limited to places with adequate preservation of foraminifera such as mid-ocean ridges and plateaus. Thus, as with most palaeoceanographic proxies, the δ18O method is best used as a complement to existing multi-proxy efforts based on diatom assemblages, HBIs and opal fluxes for unambiguous assessment of sea-ice conditions.
Assemblages and abundance patterns of radiolaria in SO sea floor sediments vary between open ocean and seasonal sea-ice zones (Lawler et al., 2021; Lowe et al., 2022), and show strong potential to develop similar indicator species and transfer approaches to infer sea-ice extent as have been done with diatoms.
Dinoflagellate cysts have been used extensively to reconstruct sea-ice extent in the Northern Hemisphere (e.g. de Vernal et al., 2005). However, similar approaches have proved less suitable in the SO where dinoflagellate assemblages are of lower diversity that in high northern latitudes (Esper and Zonneveld, 2007; Prebble et al., 2013).
3.4 Ice core proxies
Antarctic ice cores provide well-dated, high-resolution records of marine aerosols and of the isotopic composition of water transported in the atmosphere to Antarctica. Variations in the concentration of these species are linked to past changes in sea-ice conditions. Chemical tracers that have proven particularly fruitful for sea-ice reconstruction include sea salt (usually reported as sea salt sodium, ssNa), bromine (Br), methane sulfonic acid (MSA) and iodine (I). In addition to these aerosols, the stable isotope composition (δ18O) of snowfall is also influenced by sea-ice extent due to the impacts of sea ice on moisture sources conditions, air mass transport, the atmospheric hydrology and temperature over Antarctic, all of which determine δ18O in snow. Thus, in each case, sea-ice conditions alter the atmospheric conditions of the region and this is reflected in the composition of snow that is subsequently archived in the ice core (Fig. 2). However, as with all tracers in ice cores, the preservation of ssNa, Br, MSA and δ18O signals is often influenced by several factors in addition to sea ice which necessitates care in their interpretation. Despite this, the regionally integrated and higher temporal resolution information provided by ice cores complements location-specific information about the precise position of the sea-ice edge obtained from marine sediment proxies.
For the purposes of this review, we focus only on ssNa, Br and δ18O. This is because while MSA and I can be an effective tracer of sea-ice extent over decadal timescales (Thomas et al., 2019), they have not proven useful over orbital to millennial timescales due to poor signal preservation (Weller et al., 2004; Abram et al., 2013).
3.4.1 Sea-salt sodium (ssNa)
Sublimation of salty snow from the sea-ice surface is an efficient source of sea-salt aerosols over the SO according to recent field measurements (Giordano et al., 2018; Frey et al., 2020) and atmospheric modelling studies (Yang et al., 2008, 2019; Huang et al., 2018). In fact, the sea-ice surface appears to be a more important source of sea salt than bubble-bursting over the open ocean in the polar regions (Yang et al., 2008). This recognition forms the basis for interpreting ssNa in ice cores as a qualitative tracer of Antarctic WSI extent. Antarctic snow and aerosol measurements support this idea because ssNa concentrations in ice cores are typically higher in winter (relative to summer) when sea ice is expanded and an open-ocean source is further away. It is also supported by the fact that the ratio of Na+ to sulfate (SO in aerosols transported to the ice sheet is fractionated relative to seawater, characteristic of mirabilite salt precipitation in or on sea ice (Wagenbach et al., 1998; Jourdain et al., 2008). Although early studies implicated frost flower crystals as the source of this sea salt (e.g. Rankin et al., 2002), they are surprisingly difficult to break apart and entrain (Roscoe et al., 2011; Abram et al., 2013).
Coastal Antarctic ice core records with sub-annual resolution provide the opportunity to calibrate ssNa (and other chemicals) against the satellite record of recent sea-ice change. The potential for ssNa to track sea-ice changes on an annual timescale appears to be site-dependent. Some sites display a positive relationship between ssNa levels and WSI extent over recent decades (Iizuka et al., 2008; Rahaman et al., 2016; Severi et al., 2017). In contrast, other Antarctic locations show that recent variability in ssNa is linked to atmospheric pressure patterns (e.g. Fischer et al., 2004; Vance et al., 2013) while process-based modelling efforts suggest that meteorological activity (e.g. wind speed and direction) exerts a strong control on ssNa levels in ice cores over these short timescales (Levine et al., 2014; Rhodes et al., 2018). It seems likely that ssNa may become a more reliable proxy for sea ice when averaged over several years to remove the influence of meteorological conditions. In addition, the large changes in Antarctic sea-ice extent across glacial–interglacial cycles or millennial-scale climate changes are much more likely to leave an imprint on ssNa than the relatively modest recent changes.
Thus, ssNa records from Antarctic ice cores are often interpreted as regional records of changes in WSI extent over orbital timescales (Wolff et al., 2006, 2010). Comparison between the European Project for Ice Coring in Antarctica (EPICA) Dome C ssNa and a sea-ice reconstruction from a marine sediment core located in the moisture source region suggests a good level of agreement with ssNa increasing with WSI extent during glacial periods; however, the sensitivity of ssNa as a sea-ice proxy appears to weaken during peak glacial periods (Röthlisberger et al., 2010). Conversely, ssNa levels during peak interglacial periods provide valuable information on WSI variability during periods little documented in marine cores (Chadwick et al., 2022a).
An alternative interpretation of orbital to millennial ssNa variability is that it is a record of variations in the atmospheric residence time of aerosols in response to fluctuating atmospheric moisture content as temperatures rise and fall (Petit and Delmonte, 2009). Condensation-driven variations in ice core aerosol concentrations may explain the strong inverse relationship between water isotopes and both ssNa and non-sea salt Ca (nssCa) across a range of timescales (Markle et al., 2018). Although plausible, this simple theory is not yet supported by global simulations of aerosol transport changes across the last deglaciation (Reader and McFarlane, 2003; Mahowald et al., 2006). Indeed, a process-based modelling study which explicitly accounted for emission, transport and deposition of sea salt aerosol has shown that sea-ice expansion could be responsible for a substantial portion of the ssNa increase during the last glacial period relative to the Holocene in the Dome C ice core without needing to invoke meteorological changes (Levine et al., 2014). This supports the qualitative interpretation on orbital timescales. Resolution of this long-standing debate may come from three research opportunities: incorporation of sea-ice-sourced sea salt into global climate models capable of past climate simulations, development of geochemical measurements to trace the origin of sea salt in ice cores (Seguin et al., 2014), and coeval analysis of co-variability in water isotope records and marine and terrestrial aerosols (Markle et al., 2018).
3.4.2 Bromine enrichment (Brenr)
Bromine (Br), a halogen species in Antarctic ice cores, is also derived from sea salt. Its concentration in ice cores results from a complex set of photochemical reactions collectively known as bromine explosion events. These events occur over the first-year sea-ice zone during the spring months and cause the level of bromine in the atmosphere to sharply increase (Schönhardt et al., 2012). In ice cores, these events are generally recorded as bromine enrichment (Brenr) relative to the seawater Br/Na value. Over the satellite era, Brenr at Law Dome has been inversely correlated to WSI extent in the adjacent ocean (90–110∘ E) (Vallelonga et al., 2017). Over longer timescales, the Brenr record from Talos Dome shows greatest values during full interglacial periods while a depletion in bromide is observed during glacial periods (Spolaor et al., 2013). This pattern was attributed to the distance between the sea-ice edge and the ice core site with a more northerly location of the first-year sea-ice edge during glacial periods increasing the distance between the production source and the ice core site beyond the maximum distance of Br deposition observed today (Spolaor et al., 2013; Vallelonga et al., 2021). Halogens seem to be stable in ice over several tens of thousands of years, thus, alleviating temporal limitations of MSA (Vallelonga et al., 2021). However, the atmospheric chemistry of Br introduces additional complexity relative to conservative aerosol tracers such as ssNa. For example, Br can be transported inland as sea salt aerosol or gaseous compounds, which may have very different residence times in the atmosphere, therefore, impacting the ice core signal (Vallelonga et al., 2021). Further investigation is needed to fully understand and exploit the potential of this proxy.
3.4.3 Stable water isotopes (δ18O)
The stable isotope composition (δ18O) of snowfall over Antarctic is heavily influenced by sea-ice extent. This is because sea ice exerts a major control on moisture sources' locations and conditions, subsequent transport of vapour, the atmospheric circulation and vapour content and the temperature over Antarctica (which is traditionally considered the main control on δ18O). Thus sea-ice change largely determines δ18O in snow.
Noone and Simmonds (2004) and Holloway et al. (2016) demonstrated, using climate models equipped with the capability to simulate δ18O in water, that δ18O varies with sea-ice extent. They showed that less extensive sea ice permits greater transfer of heat and moisture inland and leads to less negative δ18O values. Holloway et al. (2017) provide a suite of δ18O-enabled experiments with differing sea-ice extents which help link δ18O in ice cores with sea-ice change for the LIG. These papers also demonstrated that δ18O from multiple Antarctic ice cores yield more robust information on the most likely sea-ice configuration for this climate period. More broadly, Malmierca-Vallet et al. (2018) also demonstrated that sea-ice change can be quantified from Greenland ice core δ18O measurements whilst Sime et al. (2019) showed that the intimate relationship between sea ice and ice sheet surface temperature means that δ18O in ice cores tend to provide a record that is reflective of the broadly intertwined effects of sea ice and temperature.
Thus, δ18O and other ice core approaches mentioned above have the potential advantages of providing information for all SO sectors particularly during time periods when the sea-ice edge is close to the continent (and therefore not overlying the open-ocean sediment cores). However, to be most effective, this information should always be checked (whenever possible) against the precise sea-ice information that can be gleaned from marine cores.
The C-SIDE working group (Chadwick et al., 2019; Rhodes et al., 2019) recently compiled an inventory of published marine records that provide evidence of changes in sea ice during the past 130 ka (Chadwick et al., 2022b). This compilation shows that ∼20 records represent Holocene conditions (0–12 ka ago); ∼150 records cover the LGM (ca. 21 ka ago) or part of Marine Isotope Stage 2; 14 records capture changes in sea ice back to late Marine Isotope Stage 6 (∼130 ka ago); and only two records extend beyond MIS 6 (Fig. 3). In this section, we first summarise reconstructed changes in Antarctic sea ice for the LGM, Holocene and the last interglacial period (LIG). We then consider what is known about the climate transitions between these periods.
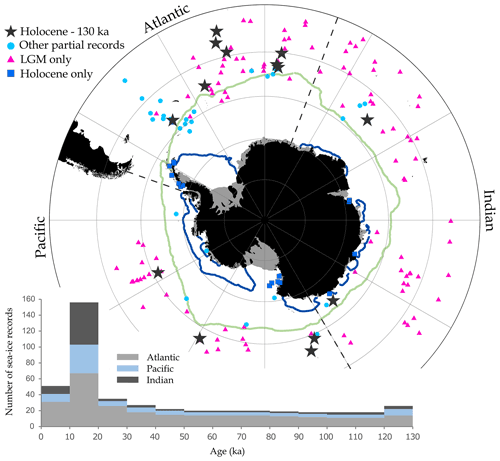
Figure 3Compilation of marine sea-ice proxy records. Map: locations of sediment cores with published sea-ice records. The 1981–2010 monthly median sea-ice extent is shown for February (blue line) and September (green line) (Fetterer et al., 2017). Plot: cumulative number of published sea-ice records vs. time from the Atlantic (light grey), Indian (dark grey) and Pacific (light blue) sectors of the Southern Ocean. (Figure adapted from Chadwick et al., 2019). The list of the cores presented herein can be found in Table S2. LGM: Last Glacial Maximum.
4.1 The Last Glacial Maximum (LGM)
The only spatially extensive attempts to map WSI and SSI extent have focused on the LGM. The first global reconstruction was completed by the “Climate: Long range Investigation, Mapping, and Prediction” Project (CLIMAP Project Members, 1981), which centred the LGM at ∼18 ka BP. This reconstruction was subsequently re-evaluated by the “Multiproxy Approach for the Reconstruction of the Glacial Ocean surface” (MARGO) project (Gersonde et al., 2005; MARGO, 2009), which used the LGM definition (19–23 ka BP) of the “Environment Processes of the Ice Age: Land, Ocean, Glaciers” (EPILOG) working group (Mix et al., 2001). Several studies have since contributed more detailed regional LGM reconstructions for the southwest Atlantic (Allen et al., 2011; Xiao et al., 2016) and Pacific (Benz et al., 2016) sectors of the SO. CLIMAP placed the austral winter and summer sea-ice edge at the faunally identified 0 ∘C winter and summer isotherm, respectively. Other studies used diatom census counts and diatom-based transfer functions.
Both CLIMAP and subsequent studies concur on the location of the WSI limit at the LGM (Fig. 4). They all suggest that WSI expanded by 5–10∘ of latitude during the LGM relative to today, leading to a LGM mean WSI surface of km2 when an equidistant projection system and a LGM Antarctic ice cap of 17×106 km2 are used (Lhardy et al., 2021). This value for WSI extent is slightly lower than previously published ( km2) using a polar stereographic projection system and a modern Antarctic ice cap for the LGM (Gersonde et al., 2005; Roche et al., 2012). The large LGM WSI cover was likely due to expansion of consolidated sea-ice area with concentrations >40 % (Crosta et al., 1998).
Unfortunately, accurate reconstruction of the LGM SSI extent is hindered by low diatom productivity at a time of very high to perennial sea-ice cover and by the lack of adequate sediment records as the SSI limit may have been above abyssal plains where preservation is poor and chronological issues are common. Recent studies suggest an expansion of the glacial SSI in the Atlantic and southwestern Pacific sectors, probably as a result of enhanced transport of sea ice from the Weddell Sea (Gersonde et al., 2005; Allen et al., 2011) and Ross Sea (Benz et al., 2016). SSI expansion was seemingly limited elsewhere (Gersonde et al., 2005), at odds with the original CLIMAP (1981) reconstructions which placed the LGM SSI limit at the modern WSI edge. However, it is long known that CLIMAP reconstructions over-estimated glacial SSI extent (Burckle et al., 1982). Based on the few control points and the modern relationship between sea ice and SST, the current understanding is that the SSI extent was 2–3 times greater (i.e. 8–12×106 km2) during the LGM when compared to today (Gersonde et al., 2005; Lhardy et al., 2021; Green et al., 2022). An important implication of this change is that the seasonal cycle of sea-ice expansion and melt was substantially greater during the LGM as compared to today with potential implications for SO and global circulation through the export of brines to the abyssal waters (Shin et al., 2003; Bouttes et al., 2010; Lhardy et al., 2021; Green et al., 2022).
Ice core records of ssNa from EDC and EDML similarly suggest that WSI reached its maximum extent between ∼27 and 18 ka BP (Wolff et al., 2006; Fischer et al., 2007) but this proxy does not provide the location of the WSI and SSI edges (Fig. 4).
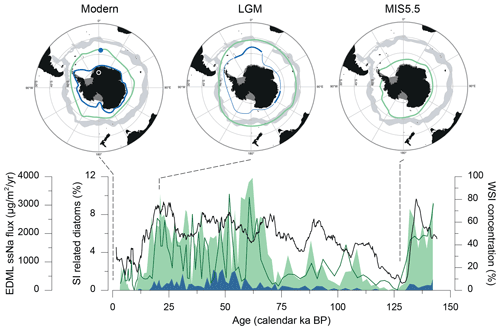
Figure 4Sea-ice reconstructions over the last glacial-interglacial period. Lower plot: ssNa flux in the EPICA Dronning Maud Land (EDML) ice core (black line) (Fischer et al., 2007, here smoothed with a 200-year running mean and plotted on the AICC2012 timescale, Veres et al., 2013). Relative abundance of sea-ice (SI) related diatoms and winter sea-ice (WSI) concentration estimates. The F. curta group (green shading) is a proxy for WSI presence while F. obliquecostata (blue shading) is a proxy for summer sea-ice (SSI) presence. WSI concentration estimates (green line) are based on the application of the MAT to diatom assemblages in marine sediment core PS1768-8 (Gersonde and Zielinski, 2000). Upper plot: maps of winter (green line) and summer (blue line) sea-ice edges in the modern (Hobbs et al., 2016), the Last Glacial Maximum (LGM at 19–23 ka BP; Gersonde et al., 2005) and the warmer-than-pre-industrial MIS5.5 (125–130 ka BP; Holloway et al., 2017). Grey field represents the modern polar front zone (Orsi et al., 1995). For the LGM map, the thick blue line indicates regions where marine data suggest the presence of SSI while the thin blue line indicates regions where SSI is inferred to be south of core sites in which SSI was not identified, and applying the modern relationship between SSI and SST to LGM SSTs (Lhardy et al., 2021). Location of marine sediment core PS1768-8 shown as a blue dot and EDML ice core shown as a white circle on the modern map.
4.2 The Holocene
The vast majority of sea-ice records covering the Holocene are located on the Antarctic continental shelves with only a handful of offshore records from the Atlantic sector of the SO (Bianchi and Gersonde, 2004; Nielsen et al., 2004; Divine et al., 2010; Xiao et al., 2016) and the southwest Pacific sector (Ferry et al., 2015a). In coastal Antarctica, diatom and HBI records generally agree at the multi-millennial timescale and allow the Holocene to be separated into three distinct periods although these periods may not be in phase around Antarctica due to regional environmental responses to long-term forcing and dating uncertainties. During the Early Holocene (∼11.5 ka to ∼8 ka BP), most coastal records suggest congruent cool surface ocean and heavy sea-ice conditions, probably in response to high glacial melt fluxes when ice sheets receded (Sjunneskog and Taylor, 2002; Heroy et al., 2008; Barbara et al., 2010; Etourneau et al., 2013; Mezgec et al., 2017; Lamping et al., 2020) because of overall warm air and ocean temperature in the SO (Masson-Delmotte et al., 2011; Shevenell et al., 2011; Totten et al., 2022). A recent analysis of seven Antarctic ice core ssNa records supports this scenario, providing evidence for heavier WSI conditions around the entire continent between 10 and 8 ka (Winski et al., 2021). The mid-Holocene (∼7 to ∼4–3 ka) was generally marked by higher SST and a well-established seasonal sea-ice cycle with a longer ice-free summer. Similarly, the EDML ice core ssNa record indicates reduced sea-ice cover in the south Atlantic sector between 6 and 5 ka, while other ice cores suggest little change in other sectors of the SO (Winski et al., 2021). The Late Holocene (∼5–3 to ∼1–0 ka) experienced a return to cool surface waters and heavy sea-ice conditions around Antarctica (Taylor et al., 2001; Sjunneskog and Taylor, 2002; Taylor and Sjunneskog, 2002; Crosta et al., 2008; Allen et al., 2010; Denis et al., 2010; Peck et al., 2015; Barbara et al., 2016; Mezgec et al., 2017; Kim et al., 2018; Q. Li et al., 2021; Totten et al., 2022). A similar increasing trend in Late Holocene sea ice is suggested by the ssNa record in the Dome Fuji ice core (Iizuka et al., 2008).
Marine offshore records from the polar front zone show a different, almost opposite, sea-ice pattern to coastal records. The Early Holocene records display high sea surface temperatures and the WSI limit is believed to have been south of 55∘ S in the Atlantic sector of the SO (Bianchi and Gersonde, 2004; Divine et al., 2010; Xiao et al., 2016). The mid-Holocene experienced a surface ocean cooling and a global re-expansion of sea ice until 3–2 ka BP. Sea ice possibly retreated again during the Late Holocene after 2 ka BP (Nielsen et al., 2004).
The long-term increase in sea ice near the coast of Antarctica has been explained by a delayed response to orbital forcing and the long memory of the SO (Renssen et al., 2005). It is also believed that increasing glacial meltwater injection into coastal surface waters as the Antarctic ice sheet retreated contributed to the rapid increase in sea-ice duration at ∼4.5–4 ka BP off Wilkes Land, East Antarctica (Ashley et al., 2021). However, the marine records published to date suggest that heavy sea-ice conditions proximal to Antarctica since ∼4 ka BP did not result in a concomitant extended sea-ice cover further from the continent. Such distinctive latitudinal variations have been attributed to the intensification of the latitudinal insolation gradient, primarily forced by obliquity and precession (Denis et al., 2010). This resulted in an intensification of the extra-tropical wind system and greater atmospheric transport toward the pole over the course of the Holocene, limiting sea-ice expansion into the SO despite a cooling world (Denis et al., 2010).
High-amplitude, decadal to millennial variations in sea-ice conditions are present throughout the Holocene. Spectral and wavelet analyses have suggested the rapid variability to be forced by solar variability (Leventer et al., 1996; Nielsen et al., 2004; Crosta et al., 2007) or driven by internal climate variability such as thermohaline circulation (Crosta et al., 2007; Debret et al., 2009) and multi-decadal to multi-centennial expression of ENSO and SAM (Etourneau et al., 2013; Pike et al., 2013; Crosta et al., 2021; Yang et al., 2021). In specific settings such as in the Mertz Glacier region off East Antarctica, the interplay between ice-sheet internal dynamics and the continental shelf seafloor may also yield centennial periodicity (Campagne et al., 2015) that may overlap with climate forcing. The lack of records covering the last 2000 years, however, limits our ability to document sea-ice variability and its drivers at a timescale relevant to the recent changes and deconvolve natural and anthropogenic forced variability over the recent decades (Thomas et al., 2019).
4.3 The Last Interglacial period (LIG)
In an effort to evaluate what a future warmer world might look like, researchers have focused on past warmer-than-present intervals. The most studied warmer-than-PI period is the LIG (129–116 ka BP) when global mean annual temperatures were ∼1–2 ∘C above PI and atmospheric CO2 concentration was similar to PI (Burke et al., 2018; Fischer et al., 2018). Reconstructions of LIG sea ice from marine sediment cores are hampered by the scarcity of records south of 60∘ S that contain LIG sediments, well-preserved sea-ice proxies and well-constrained age models. Most existing records are located north of the modern WSI extent and they show an extended period when no sea ice was present at the core sites (Chadwick et al., 2020, 2022a). It is therefore clear that the WSI extent during the LIG was substantially reduced when compared with modern conditions. Aerosol sea-ice proxies in ice cores similarly have been qualitatively interpreted as indicative of a reduced WSI cover during the LIG (Wolff et al., 2006; Spolaor et al., 2013). Due to this reduced LIG sea-ice extent, it has been difficult to accurately reconstruct the position of the WSI edge using marine core evidence alone. For this reason, there has been a focus on using δ18O from multiple Antarctic ice cores to attempt to quantify the reduction in sea ice that occurred. Using δ18O from multiple climate model experiments to deduce the relationship between δ18O and sea-ice extent, Holloway et al. (2016) and Holloway et al. (2017) calculated that the LIG WSI extent was roughly half its pre-industrial extent (Fig. 4). The calculations (based on differing δ18O in each Antarctic ice core) suggest that the reduction in WSI extent was not uniform over the SO with the greatest reductions relative to PI in the Atlantic and Indian sectors, 67 % and 59 % respectively, compared to 43 % in the Pacific sector (Holloway et al., 2017). This non-uniform WSI retreat may be related to prolonged meltwater inputs into the North Atlantic Ocean causing heat accumulation in the Southern Hemisphere with the most intense warming in the Atlantic sector (Holloway et al., 2017, 2018).
Although most marine core records of LIG WSI are located too far north to corroborate the likely LIG WSI edge location (Chadwick et al., 2020, 2022a), SST information can be used to provide additional constrain. Recent compilations of SST indicate that the SO was ∼1–3 ∘C warmer during the LIG compared to present day (Capron et al., 2014; Chadwick et al., 2020; Chandler and Langebroek, 2021b; Shukla et al., 2021). These reconstructions suggest strong LIG polar amplification when compared with middle- and low-latitude mean annual SSTs (e.g. Hoffman et al., 2017), implying reduced WSI extent in the SO. LIG warming in the SO appears to be both spatially and temporally heterogeneous, however, with the greatest SST anomalies occurring in sediment cores located near the modern subtropical front (Capron et al., 2017; Chadwick et al., 2020), and the Pacific sector seemingly reaching peak SST later than the Atlantic and Indian sectors (Chadwick et al., 2020). These spatial and temporal heterogeneities require further investigation using additional sediment cores.
Current marine and ice core data do not constrain LIG SSI. It is however very likely that there was substantially less SSI during the peak LIG warm period as compared to modern and PI. Here again, models can help constrain the SSI extent. CMIP6 models show a large spread in LIG SSI extent from 0.06 to 4.65×106 km2 and a multi-model mean (MMM) of 1.84×106 km2 (Otto-Bliesner et al., 2021), the latter being only slightly lower than the lowest SSI extent of 2.3×106 km2 recorded in February 2017 (Parkinson and DiGirolamo, 2021). We however note that none of these experiments are subjected to the prolonged meltwater inputs into the North Atlantic Ocean that preceded the LIG and that likely caused LIG heat accumulation in the Southern Hemisphere (Holloway et al., 2018). The results obtained by Otto-Bliesner et al. (2021) therefore seemingly represent an upper limit of SSI extent during the LIG peak warm period.
4.4 Transient changes – deglaciation and glaciation
The few long marine records (Crosta et al., 2004; Collins et al., 2013; Esper and Gersonde, 2014; Ferry et al., 2015a; Xiao et al., 2016; Ghadi et al., 2020; Jones et al., 2022) display sharp changes in sea ice during rapid climate transitions from cold glacial periods to warm periods (Fig. 4). These sharp changes are likely due to a quick response of sea ice to surface air temperature, SST and winds. Few records allow us to infer sea-ice dynamics across the last deglaciation and even fewer across the penultimate one (Fig. 3). Although these marine records have relatively low resolution, sea-ice retreat initiates slightly before SST increases in the same cores (depending on the baseline from which changes are inferred). In the Atlantic sector, sea-ice retreat began as early as ∼19–18 ka at 50∘ S, its northernmost extent during the LGM (Shemesh et al., 2002; Xiao et al., 2016) and reached 55∘ S by 16–15 ka (Xiao et al., 2016) (Fig. 4). In the Pacific sector, sea ice rapidly retreated at ∼20–19 ka from 56∘ S (Crosta et al., 2004; Ferry et al., 2015a) while in the Indian sector it retreated at ∼18 ka BP from 55∘ S (Ghadi et al., 2020). Additional well-dated cores are necessary to accurately document and understand the drivers of the sea-ice retreat history across deglaciations.
In contrast to the sharp changes in WSI extent inferred from the marine sediment records, ice core records show a more gradual decline in ssNa across the last deglaciation starting from 19 ka (Röthlisberger et al., 2004) (Fig. 4). This is likely because the ssNa flux is integrating a signal from a relatively wide area relative to marine sediment records that respond to sea-ice changes at their specific location. Additionally, ssNa may also be influenced by other complicating factors discussed in Sect. 3.4.1. Interestingly, ice core records suggest that the ssNa responded in phase with Antarctic air temperature, leading CO2 concentrations by around 500 years over the last glacial cycle (Bauska et al., 2021). Given that Antarctic air temperature led CO2 concentrations during the last deglaciation (Marcott et al., 2014), one could infer that sea-ice change also led CO2 increase. If the phasing can be verified, it would suggest that Antarctic sea ice exerted a strong control on atmospheric CO2 concentration changes through its role on global ocean circulation (Gildor and Tziperman, 2000; Ferrari et al., 2014; Marzocchi and Jansen, 2019; Stein et al., 2020).
Although very few records cover the last climatic cycle (Fig. 3), marine proxy-based sea-ice records show increases in sea-ice cover during cold periods of the last glacial cycle with small expansions during MIS 5 stadial periods and the first important re-advance during MIS 4 (Gersonde and Zielinski, 2000; Crosta et al., 2004; Kohfeld and Chase, 2017; Nair et al., 2019) (Fig. 4). This contrasts with the gradual increase in ssNa documented by ice core records (Wolff et al., 2006) which would suggest a progressive sea-ice expansion from the last glacial inception (∼116 ka BP) to the last glacial (∼20 ka BP). The global and inter-basinal differences in sea-ice changes over the glaciation, are developed in Chadwick et al. (2022b).
In each section, we have highlighted the progress, assumptions and limitations of each of the sea-ice proxies used to reconstruct different aspects of Antarctic sea ice. Our focus in the following section is not on the future work needed to advance each proxy on its own but rather how the records (and proxies) can be used together to help fill the existing spatio-temporal gaps.
The inventory of sea-ice records described above provides a first step in understanding what records are currently available but also highlights major spatial and temporal gaps, and the challenges of developing a comprehensive understanding of past changes in Antarctic sea-ice extent. First, the spatial distribution of these samples reveals several gaps with ocean sampling limited by preservation issues in deep ocean basins. As a result, samples are clustered along ridges, plateaus and coastal settings, and this distribution has limited our ability to develop latitudinal transects and document the dynamic of sea-ice retreat at deglaciations and sea-ice advance during glacial inceptions. Furthermore, coastal sites around Antarctica are limited to the Holocene time period because the expansion of the Antarctic ice cap over the continental shelf during the peak glaciation has eroded sediments deposited during preceding interglacial periods. As such, only sites beyond the continental shelf can be used to reconstruct Antarctic sea-ice extent beyond the Holocene.
One important next step in improving our representation of Antarctic sea-ice changes over glacial–interglacial timescales involves the development of multi-proxy approaches. These approaches could involve (a) combining marine and ice core indicators of sea-ice change, (b) combining multiple sea-ice indicators from marine sediments and (c) integrating data with model simulations. The first approach allows for comparison of open ocean reconstructions and long-term records from Antarctica but requires a recognition of differences in the spatial representation and processes controlling sea-ice proxies in these environments. Information from marine sediment cores tend to be representative of regional conditions and, as a result, many records are necessary to draw robust interpretations at the basin scale. In contrast, materials archived in ice cores are generally representative of a much larger spatial area and, thus, can complement marine-based reconstructions. However, ice core proxies represent an integration of oceanic and atmospheric processes, produce only qualitative reconstructions of sea-ice changes, and the signal may become saturated during glacial periods. Combining qualitative and quantitative proxies using a range of reconstruction techniques and archives will provide useful and complementary insights, but producing consistent and coherent interpretations of past time periods from such disparate records remains a challenge.
The second potential step forward involves integrating multiple lines of evidence from marine sediment archives themselves. To date, most Antarctic marine-based sea-ice records are inferred from diatom assemblages. Where sediment preservation allows it, the incorporation of planktonic/benthic foraminiferal oxygen isotope reconstructions from down core records has the potential to provide complementary insights into changes in the cold “winter water” formed below WSI. Importantly, thoughtful integration of multiple proxies also has the potential to expand the aerial coverage of our knowledge of sea-ice changes. The current methods of applying transfer functions to reconstruct sea-ice conditions at open ocean sites have been used for many decades but their robustness drops along the Antarctic continental shelf where the signal–to–noise ratio (i.e. high variability in diatom assemblages despite low ranges of surface conditions) decreases. Other proxies such as HBIs, ancient DNA (De Schepper et al., 2019) or geochemical proxies in mumiyo, the fossilised stomach oil of snow petrels (McClymont et al., 2022), may complement diatom assemblage reconstructions especially in the coastal regions. When compared to microfossil assemblages and/or other sea-ice indicators from ice cores, these organic proxies may contribute valuable information on the feedback mechanisms between sea ice and ice shelves because of their potential to reconstruct sea-ice conditions proximal to ice shelves where the preservation of diatoms is often affected by opal dissolution. However, these new tools still need to be calibrated to provide quantitative sea-ice concentration values as has been initiated around the Antarctic Peninsula (Vorrath et al., 2019; Lamping et al., 2021). Ultimately, improved spatial and temporal coverage of near-coastal sea-ice conditions for pre-Holocene time intervals such as the last deglaciation and the Last Interglacial period is critical for elucidating the impact of sea-ice variability on ice-shelf dynamics.
The third approach is to combine discrete marine records of Antarctic sea ice with climate model simulations to provide a fuller picture of changes in sea-ice cover during past time periods. These type of approaches tend to fall into three distinct categories or strands of work: (i) the use climate model output, including from proxy-enabled models, to help interpret measurements from marine and ice cores; (ii) the use of marine and ice core based sea-ice reconstructions to investigate past transient changes and their underlying processes; and (iii) the assimilation of data into models to help provide better records and an improved understanding of past sea-ice changes.
The first of these strands of model–data work proved valuable in improving our knowledge on the spatial distribution of WSI and SSI fields at the LGM (Lhardy et al., 2021; Green et al., 2022) and the LIG (Holloway et al., 2016, 2017), and on the associated impacts on the global ocean circulation and carbon cycle (Marzocchi et al., 2017).
On the second strand, using transient simulations, sea-ice evolution has been investigated for the last glacial period with coupled models (Menviel et al., 2015) and for the whole 130 ka through models with simplified ocean and sea-ice dynamics (Brovkin et al., 2012; Stein et al., 2020). However, Antarctic sea ice has not been studied in detail therein and simulations were generally restricted to the total sea-ice extent with little comparison to marine or ice core records of sea ice. Additional transient climate simulations of parts of the last glacial–interglacial cycle, which can provide estimates of the Antarctic sea-ice evolution exist (e.g. Menviel et al., 2012, 2018; Bagniewski et al., 2017; Yeung et al., 2019) but have not been exploited to date. A thorough model–data comparison of the transient changes in Antarctic sea ice over the last glacial–interglacial cycle would improve our understanding of the interaction between changes in climate, sea ice and ocean circulation. In this vein, Holloway et al. (2018) tested limited-length CMIP-model (2000 year) transient simulations focused on the LIG against ice and marine core based reconstructions of SST and sea ice from the SO. This approach suggested that the H11 event, interrupting the penultimate deglaciation, could explain the SO sea-ice minima and SST maximum recorded at 127 ka even though some PMIP4 experiments similarly simulate a reduced LIG sea-ice extent under LIG-only boundary conditions (Otto-Bliesner et al., 2021; Yeung et al., 2021).
On a third strand, quantitative sea-ice data can also be assimilated into models to direct their trajectories as done recently for the last 2000 years (Crosta et al., 2021). In return, models can provide spatial links to address the geographic gaps that hamper a transect-style approach based solely on the marine and ice core records. Such an approach will facilitate documentation of the dynamics of sea-ice changes over the last glacial cycle and, especially, will help resolve the speed of sea-ice retreat during deglaciations and the speed of advance during glacial inceptions. It will also improve our understanding of the factors driving the differences evident between ocean basins (Chadwick et al., 2022b). Finally, this approach will help refine our understanding of the impact of Antarctic sea ice on the global ocean circulation and carbon cycle through time.
Data associated with Table S2 can be found at https://doi.org/10.1594/PANGAEA.943275 (Chadwick et al., 2022c).
The supplement related to this article is available online at: https://doi.org/10.5194/cp-18-1729-2022-supplement.
CX, KEK, HCB conceptualised the project and led the writing of the original draft. CX, KEK, HCB, MC, ADV, OE, JE, AL, JM, RHR participated to the writing of the original draft. CX, MC, JE and JP produced the figures. CX and MC produced the tables. MC, JJ, KAL and JGP participated to the curation of the data. All authors contributed to the improvement and finalisation of the manuscript through several iterations.
The contact author has declared that none of the authors has any competing interests.
Publisher’s note: Copernicus Publications remains neutral with regard to jurisdictional claims in published maps and institutional affiliations.
This article is part of the special issue “Reconstructing Southern Ocean sea-ice dynamics on glacial-to-historical timescales”. It is not associated with a conference.
This work was conducted as part of Phase 1 of the Cycles of Sea-Ice Dynamics in the Earth system (C-SIDE) Past Global Changes (PAGES) scientific working group; this paper benefited from discussions with participants in two C-SIDE workshops. We thank Maggie Duncan for her help with data curation. We thank Niccolò Maffezzoli, Andrés S. Rigual Hernández and one anonymous reviewer, as well as the editor Bjorg Risebrobakken, for their constructive comments.
This work was funded by a PAGES data stewardship grant to Matthew Chadwick (DSS_105; funding to work on the data inventory) and a Canadian Natural Sciences and Engineering Research Council (Discovery grant RGPIN342251) to KEK (which funded the initial workers on the inventory and a lot of the people who attended C-SIDE workshops). Alice du Vivier acknowledges support through the National Center for Atmospheric Research which is sponsored by the National Science Foundation under Cooperative agreement (grant no. 1852977). Katrin J. Meissner and Laurie Menviel acknowledge funding from the Australian Research Council (grant nos. FT180100606 and DP180100048). Kelly-Anne Lawler is supported by an Australian Research Training Program (RTP) scholarship. Carina Lange acknowledges partial support from IDEAL Research Center (grant no. 15150003). Juliane Müller, Nele Lamping and Maria-Elena Vorrath were funded through a Helmholtz Association grant (grant no. VH-NG-1101). Abhilash Nair acknowledges the Director, ESSO-NCPOR, Ministry of Earth Sciences, India for facilitating the Antarctic palaeo sea-ice research in NCPOR. Christina Riesselman acknowledges support from the New Zealand Ministry of Business, Innovation and Employment Antarctic Science Platform (grant no. ANTA1801). Joseph G. Prebble was funded by GNS Science SSIF funding via the Global Change Through Time Programme.
This paper was edited by Bjørg Risebrobakken and reviewed by Andres Rigual-Hernandez, Niccolò Maffezzoli and one anonymous referee.
Abernathey, R. P., Cerovecki, I., Holland, P. R., Newsom, E., Mazloff, M., and Talley, L. D.: Water-mass transformation by sea ice in the upper branch of the Southern Ocean overturning, Nat. Geosci., 9, 596–601, 2016.
Abram, N. J., Wolff, E. W., and Curran, M. A. J.: A review of sea ice proxy information from polar ice cores, Quaternary Sci. Rev., 79, 168–183, 2013.
Ackley, S. F.: A review of sea-ice weather relationships in the Southern Hemisphere, in: Proceeding of the Canberra Symposium: Sea Level, Ice and Climatic Change, edited by: Allison, I., IAHS Publication, Guildfold, UK, Vol. 131, 127–159, 1980.
Ainley, D., Woehler, E. J., and Lescroël, A.: Birds and Antarctic sea ice, in: Sea Ice, Third Edition, edited by: Thomas, D. N., Wiley-Blackwell, Oxford, UK, 570–582, 2017.
Alkama, R., Koffi, E. N., Vavrus, S. J., Diehl, T., Francis, J. A., Stroeve, J., Forzieri, G., Vihma, T., and Cescatti, A.: Wind amplifies the polar sea ice retreat, Environ. Res. Lett., 15, 124022, https://doi.org/10.1088/1748-9326/abc379, 2020.
Allen, C. S., Oakes-Fretwell, L., Anderson, J. B., and Hodgson, D. A.: A record of Holocene glacial and oceanographic variability in Neny Fjord, Antarctic Peninsula, Holocene, 20, 551–564, 2010.
Allen, C. S., Pike, J., and Pudsey, C. J.: Last glacial–interglacial sea-ice cover in the SW Atlantic and its potential role in global deglaciation, Quaternary Sci. Rev., 30, 2446–2458, 2011.
Ancel, A., Kooyman, G. L., Ponganis, P. J., Gendner, J. P., Lignon, J., Mestre, X., Huin, N., Thorson, P. H., Robisson, P., and Le Maho, Y.: Foraging behaviour of emperor penguins as a resource detector in winter and summer, Nature, 360, 336–339, 1992.
Andreas, E. L. and Ackley, S. F.: On the differences in ablation seasons of Arctic and Antarctic sea ice, J. Atmos. Sci., 39, 440–447, 1982.
Armand, L., Ferry, A., and Leventer, A.: Ch. 26. Advances in palaeo sea-ice estimation, in: Sea Ice Edition 3, edited by: Thomas, D., Wiley-Blackwell, Oxford, UK, 600–629, 2017.
Armand, L. K., Crosta, X., Romero, O., and Pichon, J.-J.: The biogeography of major diatom taxa in Southern Ocean sediments: 1. Sea ice related species, Palaeogeogr. Palaeoclim. Palaeoecol., 223, 93–126, 2005.
Arrigo, K. R., Mills, M. M., Kropuenske, L. R., van Dijken, G. L., Alderkamp, A.-C., and Robinson, D. H.: Photophysiology in two major Southern Ocean phytoplankton taxa: Photosynthesis and growth of Phaeocystis antarctica and Fragilariopsis cylindrus under different irradiance levels, Integr. Compar. Biol., 50, 950–966, 2010.
Arrigo, K. R. and van Dijken, G. L.: Phytoplankton dynamics within 37 Antarctic coastal polynya systems, J. Geophys. Res.-Oceans, 108, 3271, https://doi.org/10.1029/2002JC001739, 2003.
Arrigo, K. R. and van Dijken, G. L.: Interannual variation in air-sea CO2 flux in the Ross Sea, Antarctica: A model analysis, J. Geophys. Res., 112, C03020, https://doi.org/10.1029/2006JC003492, 2007.
Arrigo, K. R., van Dijken, G., and Pabi, S.: Impact of a shrinking Arctic ice cover on marine primary production, Geophys. Res. Lett., 35, L19603, https://doi.org/10.1029/2008GL035028, 2008.
Arrigo, K. R., van Dijken, G. L., and Strong, A. L.: Environmental controls of marine productivity hot spots around Antarctica, J. Geophys. Res.-Oceans, 120, 5545–5565, 2015.
Arzel, O., Fichefet, T., and Goosse, H.: Sea ice evolution over the 20th and 21st centuries as simulated by current AOGCMs, Ocean Modell., 12, 401–415, 2006.
Ashley, K. E., McKay, R., Etourneau, J., Jimenez-Espejo, F. J., Condron, A., Albot, A., Crosta, X., Riesselman, C., Seki, O., Massé, G., Golledge, N. R., Gasson, E., Lowry, D. P., Barrand, N. E., Johnson, K., Bertler, N., Escutia, C., Dunbar, R., and Bendle, J. A.: Mid-Holocene Antarctic sea-ice increase driven by marine ice sheet retreat, Clim. Past, 17, 1–19, https://doi.org/10.5194/cp-17-1-2021, 2021.
Bagniewski, W., Meissner, K. J., and Menviel, L.: Exploring the oxygen isotope fingerprint of Dansgaard-Oeschger variability and Heinrich events, Quaternary Sci. Rev., 159, 1–14, 2017.
Barbara, L., Crosta, X., Leventer, A., Schmidt, S., Etourneau, J., Domack, E., and Massé, G.: Environmental responses of the Northeast Antarctic Peninsula to the Holocene climate variability, Paleoceanography, 31, 131–147, 2016.
Barbara, L., Crosta, X., Massé, G., and Ther, O.: Deglacial environments in eastern Prydz Bay, East Antarctica, Quaternary Sci. Rev., 29, 2731–2740, 2010.
Bauska, T. K., Marcott, S. A., and Brook, E.J. : Abrupt changes in the global carbon cycle during the last glacial period, Nat. Geosci., 14, 91–96, 2021.
Bayer-Giraldi, M., Uhlig, C., John, U., Mock, T., and Valentin, K.: Antifreeze proteins in polar sea ice diatoms : Diversity and gene expression in the genus Fragilariopsis, Environ. Microbiol., 12, 1041–1052, 2010.
Belt, S. T.: Source-specific biomarkers as proxies for Arctic and Antarctic sea ice, Org. Geochem., 125, 277–298, 2018.
Belt, S. T., Brown, T. A., Smik, L., Tatarek, A., Wiktor, J., Stowasser, G., Assmy, P., Allen, C. S., and Husum, K.: Identification of C25 highly branched isoprenoid (HBI) alkenes in diatoms of the genus Rhizosolenia in polar and sub-polar marine phytoplankton, Org. Geochem., 110, 65–72, 2017.
Belt, S. T., Smik, L., Brown, T. A., Kim, J. H., Rowland, S. J., Allen, C. S., Gal, J. K., Shin, K. H., Lee, J. I., and Taylor, K. W. R.: Source identification and distribution reveals the potential of the geochemical Antarctic sea ice proxy IPSO25, Nat. Commun., 7, 12655, https://doi.org/10.1038/ncomms12655, 2016.
Benz, V., Esper, O., Gersonde, R., Lamy, F., and Tiedemann, R.: Last Glacial Maximum sea surface temperature and sea-ice extent in the Pacific sector of the Southern Ocean, Quaternary Sci. Rev., 146, 216–237, 2016.
Bianchi, C. and Gersonde, R.: Climate evolution at the last deglaciation: The role of the Southern Ocean, Earth Planet. Sci. Lett., 228, 407–424, 2004.
Bintanja, R., van Oldenborgh, G. J., Drijfhout, S. S., Wouters, B., and Katsman, C. A.: Important role for ocean warming and increased ice-shelf melt in Antarctic sea-ice expansion, Nat. Geosci., 6, 376–379, 2013.
Bintanja, R., van Oldenborgh, G. J., and Katsman, C. A.: The effect of increased fresh water from Antarctic ice shelves on future trends in Antarctic sea ice, Ann. Glaciol., 56, 120–126, https://doi.org/10.3189/2015AoG69A001, 2015.
Blockley, E., Vancoppenolle, M., Hunke, E., Bitz, C., Feltham, D., Lemieux, J.-F., Losch, M., Maisonnave, E., Notz, D., Rampal, P., Tietsche, S., Tremblay, B., Turner, A., Massonnet, F., Olason, E., Roberts, A., Aksenov, Y., Fichefet, T., Garric, G., Iovino, D., Madec, G., Rousset, C., Salas y Melia, D., and Schroeder, D.: The future of sea ice modeling: Where do we go from here?, BAMS Meeting Summary, https://doi.org/10.1175/BAMS-D-20-0073.1, 2020.
Bouttes, N., Paillard, D., and Roche, D. M.: Impact of brine-induced stratification on the glacial carbon cycle, Clim. Past, 6, 575–589, https://doi.org/10.5194/cp-6-575-2010, 2010.
Bracegirdle, T. J., Connolley, W. M., and Turner, J.: Antarctic climate change over the twenty first century, J. Geophys. Res.-Atmos., 113, D03103, https://doi.org/10.1029/2007JD008933, 2008.
Brovkin, V., Ganopolski, A., Archer, D., and Munhoven, G.: Glacial CO2 cycle as a succession of key physical and biogeochemical processes, Clim. Past, 8, 251–264, https://doi.org/10.5194/cp-8-251-2012, 2012.
Burke, K. D., Williams, J. D., Chandler, M. A., Haywood, A. M., Lunt, D. J., and Otto-Bliesner, B. L.: Pliocene and Eocene provide best analogs for near-future climates, P. Natl. Acad. Sci. USA, 115, 13288–13293, 2018.
Burckle, L. H.: Diatom dissolution patterns in sediments of the Southern ocean, Geol. Soc. Am. J., 15, 536–537, 1983.
Burckle, L.H. and Cirilli, J.: Origin of Diatom Ooze Belt in the Southern Ocean: Implications for Late Quaternary Paleoceanography, Micropaleontology, 33, 82–86, 1987.
Burckle, L. H., Jacob, S. S., and McLaughlin, R. B.: Late austral spring diatom distribution between New Zealand and the Ross Ice Shelf, Antarctica: Hydrography and sediment correlations, Micropaleontology, 33, 74–81, 1987.
Burckle, L. H. and Mortlock, R.: Sea-ice extent in the Southern Ocean during the Last Glacial Maximum: another approach to the problem, Ann. Glaciol., 27, 302–304, 1998.
Burckle, L. H., Robinson, D., and Cooke, D.: Reappraisal of sea-ice distribution in Atlantic and Pacific sectors of the Southern Ocean at 18,000 yr BP, Nature, 299, 435–437, 1982.
Cabedo-Sanz, P., Smik, L., and Belt, S. T.: On the stability of various highly branched isoprenoid (HBI) lipids in stored sediments and sediment extracts, Org. Geochem., 97, 74–77, 2016.
Campagne, P., Crosta, X., Houssais, M. N., Swingedouw, D., Schmidt, S., Martin, A., Devred, E., Capo, S., Marieu, V., Closset, I., and Massé, G.: Glacial ice and atmospheric forcing on the Mertz Glacier Polynya over the past 250 years, Nat. Commun., 6, 6642, https://doi.org/10.1038/ncomms7642, 2015.
Capron, E., Govin, A., Stone, E. J., Masson-Delmotte, V., Mulitza, S., Otto-Bliesner, B., Rasmussen, T. L., Sime, L. C., Waelbroeck, C., and Wolff, E. W.: Temporal and spatial structure of multi-millennial temperature changes at high latitudes during the Last Interglacial, Quaternary Sci. Rev., 103, 116–133, 2014.
Capron, E., Govin, A., Feng, R., Otto-Bliesner, B. L., and Wolff, E. W.: Critical evaluation of climate syntheses to benchmark CMIP6/CMIP4 127 k Last Interglacial simulations in the high-latitude regions, Quaternary Sci. Rev., 168, 137–150, https://doi.org/10.1016/j.quascirev.2017.04.019, 2017.
Cavalieri, D. J. and Parkinson, C. L.: Antarctic sea ice variability and trends, 1979–2006, J. Geophys. Res., 113, C07004, https://doi.org/10.1029/2007JC004564, 2008.
Cavalieri, D. J., Parkinson, C. L., and Vinnikov, K. Y.: 30-Year satellite record reveals contrasting Arctic and Antarctic decadal sea ice variability, Geophys. Res. Lett., 30, 1970, https://doi.org/10.1029/2003GL018031, 2003.
Chadwick, M., Jones, J., Lawler, K.-A., Prebble, J., Kohfeld, K. E., and Crosta, X.: Understanding glacial-interglacial changes in Southern Ocean sea ice, PAGES Magazine, 27, 86, https://doi.org/10.22498/pages.27.2.86, 2019.
Chadwick, M., Allen, C. S., Sime, L. C., and Hillenbrand, C. D.: Analysing the timing of peak warming and minimum winter sea-ice extent in the Southern Ocean during MIS 5e, Quaternary Sci. Rev., 229, 106134, https://doi.org/10.1016/j.quascirev.2019.106134, 2020.
Chadwick, M., Allen, C. S., Sime, L. C., Crosta, X., and Hillenbrand, C.-D.: Reconstructing Antarctic winter sea-ice extent during Marine Isotope Stage 5e, Clim. Past, 18, 129–146, https://doi.org/10.5194/cp-18-129-2022, 2022a.
Chadwick, M., Crosta, X., Esper, O., Thöle, L., and Kohfeld, K. E.: Compilation of Southern Ocean sea-ice records covering the last glacial-interglacial cycle (12–130 ka), Clim. Past Discuss. [preprint], https://doi.org/10.5194/cp-2022-15, in review, 2022b.
Chadwick, M., Crosta, X., Esper, O., and Kohfeld, K. E.: Southern Ocean marine sediment core sea-ice records for the last 150,000 years, PANGAEA [data set], https://doi.org/10.1594/PANGAEA.943275, 2022c.
Chandler, D. and Langebroek, P.: Southern Ocean sea surface temperature synthesis: Part 1. Evaluation of temperature proxies at glacial-interglacial time scales, Quaternary Sci. Rev., 271, 107191, https://doi.org/10.1016/j.quascirev.2021.107191, 2021a.
Chandler, D. and Langebroek, P.: Southern Ocean sea surface temperature synthesis: Part 2. Penultimate glacial and last interglacial, Quaternary Sci. Rev., 271, 107190, https://doi.org/10.1016/j.quascirev.2021.107190, 2021b.
Chase, Z., Kohfeld, K., and Matsumoto, K.: Controls on rates of opal burial in the Southern Ocean, Global Biogeochem. Cy., 29, 1599–1616, 2015.
Ciasto, L. M., Simpkins, G. R., and England, M. H.: Teleconnections between Tropical Pacific SST Anomalies and Extratropical Southern Hemisphere Climate, J. Climate, 28, 56–65, 2015.
CLIMAP Project Members: Seasonal reconstructions of the Earth’s surface at the last glacial maximum, Geological Society of America Map and Chart Series, MC-36, Lamont-Doherty Geological Observatory, Columbia University, Palisades NY, USA, 1981.
Collins, L. G., Allen, C. S., Pike, J., Hodgson, D. A., Weckström, K., and Massé, G.: Evaluating highly branched isoprenoid (HBI) biomarkers as a novel Antarctic sea-ice proxy in deep ocean glacial age sediments, Quaternary Sci. Rev., 79, 87–98, 2013.
Comiso, J. C.: Large-scale characteristics and variability of the global sea ice cover, in: Sea Ice: An Introduction to its Physics, Chemistry, Biology and Geology, edited by: Thomas, D. N. and Dieckmann, G., Wiley-Blackwell, Oxford, UK, 112–142, 2003.
Comiso, J. C., Gersten, R. A., Stock, L. V., Turner, J., Perez, G. J., and Cho, K.: Positive trend in the Antarctic sea ice cover and associated changes in surface temperature, J. Climate, 30, 2251–2267, https://doi.org/10.1175/JCLI-D-16-0408.1, 2017.
Cooke, D. W. and Hays, J. D.: Estimates of Antarctic ocean seasonal ice-cover during glacial intervals, in: Antarctic Geoscience, edited by: Craddock, C., University of Wisconsin Press, Madison, WI, USA, 1017–1025, 1982.
Crosta, X., Debret, M., Denis, D., Courty, M. A., and Ther, O.: Holocene long- and short-term climate changes off Adélie Land, East Antarctica, Geochem. Geophys. Geosyst., 8, Q11009, https://doi.org/10.1029/2007GC001718, 2007.
Crosta, X., Denis, D., and Ther, O.: Sea ice seasonality during the Holocene, Adélie Land, East Antarctica, Mar. Micropaleontol., 66, 222–232, 2008.
Crosta, X., Etourneau, J., Orme, L., Dalaiden, Q., Campagne, P., Swingedouw, D., Goosse, H., Massé, G., Miettinen, A., McKay, R.M., Dunbar, R.B., Escutia, C., and Ikehara, M.: Multi-decadal trends in Antarctic sea-ice extent driven by ENSO-SAM over the last 2,000 years, Nat. Geosci., 14, 156–160, 2021.
Crosta, X., Pichon, J.-J., and Burckle, L. H.: Application of modern analog technique to marine Antarctic diatoms: Reconstruction of maximum sea-ice extent at the Last Glacial Maximum, Paleoceanography, 13, 284–297, 1998.
Crosta, X., Romero, O., Armand, L. K., and Pichon, J.-J.: The biogeography of major diatom taxa in Southern Ocean sediments: 2. Open ocean related species, Palaeogeogr. Palaeoclimatol. Palaeoecol., 223, 66–92, 2005.
Crosta, X., Sturm, A., Armand, L., and Pichon, J.-J.: Late Quaternary sea ice history in the Indian sector of the Southern Ocean as recorded by diatom assemblages, Mar. Micropaleontol., 50, 209–223, 2004.
Deb, P., Dash, M. K., and Pandey, P. C.: Effect of Pacific warm and cold events on the sea ice behavior in the Indian sector of the Southern Ocean, Deep Sea Res. Part I, 84, 59–72, 2014.
Debret, M., Sebag, D., Crosta, X., Massei, N., Petit, J. R., Chapron, E., and Bout-Roumazeilles, V.: Evidence from wavelet analysis for a mid-Holocene transition in global climate forcing, Quaternary Sci. Rev., 28, 2675–2688, 2009.
DeFelice, D. R.: Relative diatom abundance as tool for monitoring winter sea ice fluctuations in southeast Atlantic, Ant. J. United States, 14, 105–106, 1979a.
DeFelice, D. R.: Surface Lithofacies, Biofacies, and Diatom Diversity Patterns as Models for Delineation of Climatic Change in the Southeast Atlantic Ocean, Ph.D., Geology, Florida State University, Tallahassee, FL, USA, 209 pp., 1979b.
DeJong, H. B. and Dunbar, R. B.: Air-Sea CO2 exchange in the Ross Sea, Antarctica, J. Geophys. Res.-Oceans, 122, 8167–8181, 2017.
Delworth, T. L. and Zeng, F.: Simulated impact of altered Southern Hemisphere winds on the Atlantic Meridional Overturning Circulation, Geophys. Res. Lett., 35, L20708, https://doi.org/10.1029/2007GC001718, 2008.
Denis, D., Crosta, X., Barbara, L., Massé, G., Renssen, H., Ther, O., and Giraudeau, J.: Sea ice and wind variability during the Holocene in East Antarctica: insight on middle–high latitude coupling, Quaternary Sci. Rev., 29, 3709–3719, 2010.
De Schepper, S., Ray, J. L., Sandness Skaar, K., Ijaz, U. Z., Stein, R., and Larsen, A.: The potential of sedimentary ancient DNA for reconstructing past sea ice evolution, The ISME J., 13, 2566–2577, 2019.
de Vernal, A., Eynaud, F., Henry, M., Hillaire-Marcel, C., Londeix, L., Mangin, S., Matthiessen, J., Marret, F., Radi, T., Rochon, A., Solignac, S., and Turon, J.-L.: Reconstruction of sea-surface conditions at middle to high latitudes of the Northern Hemisphere during the Last Glacial Maximum (LGM) based on dinoflagellate cyst assemblages, Quaternary Sci. Rev., 24, 897–924, 2005.
Dieckmann, G., Rohardt, G., Hellmer, H., and Kipfstuhl, J.: The occurrence of ice platelets at 250 m depth near the Filchner Ice Shelf and its significance for sea ice biology, Deep Sea Res. Part A, 33, 141–148, 1986.
Di Tullio, G. R., Grebmeier, J. M., Arrigo, K. R., Lizotte, M. P., Robinson, D. H., Leventer, A., Barry, J. P., VanWoert, M. L., and Dunbar, R. R.: Rapid and early export of Phaeocystis antarctica blooms in the Ross Sea, Antarctica, Nature, 404, 595–598, 2000.
Divine, D. V., Koç, N., Isaksson, E., Nielsen, S., Crosta, X., and Godtliebsen, F.: Holocene Antarctic climate variability from ice and marine sediment cores: Insights on ocean-atmosphere interaction, Quaternary Sci. Rev., 29, 303–312, 2010.
Doddridge, E. W. and Marshall, J.: Modulation of the seasonal cycle of Antarctic sea ice extent related to the Southern Annular Mode, Geophys. Res. Lett., 44, 9761–9768, 2017.
Eayrs, C., Li, X., Raphael, M. N., and Holland, D. M.: Rapid decline in Antarctic sea ice in recent years hints at future change, Nat. Geosci., 14, 460–464, https://doi.org/10.1038/s41561-021-00768-3, 2021.
Eicken, H.: The role of sea ice in structuring Antarctic ecosystems, Polar Biol., 12, 3–13, 1992.
England, M. R., Polvani, L., Sun, L., and Deser, C.: Tropical response to projected declined in Arctic and Antarctic sea-ice loss, Nat. Geosci., 13, 275–281, 2020.
Esper, O. and Gersonde, R.: New tools for the reconstruction of Pleistocene Antarctic sea ice, Palaeogeogr. Palaeoclimatol. Palaeoecol., 399, 260–283, 2014.
Esper, O., Gersonde, R., and Kadagies, N.: Diatom distribution in southeastern Pacific surface sediments and their relationship to modern environmental variables, Palaeogeogr. Palaeoclimatol. Palaeoecol., 287, 1–27, 2010.
Esper, O. and Zonneveld, K. A. F.: The potential of organic-walled dinoflagellate cysts for the reconstruction of past sea-surface conditions in the Southern Ocean, Mar. Micropaleontol., 65, 185–212, 2007.
Etourneau, J., Collins, L. G., Willmott, V., Kim, J.-H., Barbara, L., Leventer, A., Schouten, S., Sinninghe Damsté, J. S., Bianchini, A., Klein, V., Crosta, X., and Massé, G.: Holocene climate variations in the western Antarctic Peninsula: evidence for sea ice extent predominantly controlled by changes in insolation and ENSO variability, Clim. Past, 9, 1431–1446, https://doi.org/10.5194/cp-9-1431-2013, 2013.
Fan, T., Deser, C., and Schneider, D. P.: Recent Antarctic sea ice trends in the context of Southern Ocean surface climate variations since 1950, Geophys. Res. Lett., 41, 2419–2426, 2014.
Ferrari, R., Jansen, M. F., Adkins, J. F., Burke, A., Stewart, A. L., and Thompson, A. F.: Antarctic sea ice control on ocean circulation in present and glacial times, P. Natl. Acad. Sci. USA, 111, 8753–8758, 2014.
Ferreira, D., Marshall, J., Bitz, C. M., Solomon, S., and Plumb, A.: Antarctic Ocean and Sea Ice Response to Ozone Depletion: A Two-Time-Scale Problem, J. Climate, 28, 1206–1226, 2015.
Ferry, A. J., Crosta, X., Quilty, P. G., Fink, D., Howard, W., and Armand, L. K.: First records of winter sea ice concentration in the southwest Pacific sector of the Southern Ocean, Paleoceanography, 30, 1525–1539, 2015a.
Ferry, A. J., Prvan, T., Jersky, B., Crosta, X., and Armand, L. K.: Statistical modeling of Southern Ocean marine diatom proxy and winter sea ice data: Model comparison and developments, Prog. Oceanogr., 131, 100–112, 2015b.
Fetterer, F., Knowles, K., Meier, W. N., Savoie, M., and Windnagel, A. K.: Sea Ice Index, National Snow and Ice Data Center, https://doi.org/10.7265/N5K072F8, Boulder, Colorado, USA, 2017 (updated daily).
Fischer, H., Fundel, F., Ruth, U., Twarloh, B., Wegner, A., Udisti, R., Becagli, S., Castellano, E., Morganti, A., Severi, M., Wolff, E., Littot, G., Röthlisberger, R., Mulvaney, R., Hutterli, M. A., Kaufmann, P., Federer, U., Lambert, F., Bigler, M., Hansson, M., Jonsell, U., de Angelis, M., Boutron, C., Siggaard-Andersen, M.-L., Steffensen, J. P., Barbante, C., Gaspari, V., Gabrielli, P., and Wagenbach, D.: Reconstruction of millennial changes in dust emission, transport and regional sea ice coverage using the deep EPICA ice cores from the Atlantic and Indian Ocean sector of Antarctica, Earth Planet. Sci. Lett. 260, 340–354, 2007.
Fischer, H., Meissner, K. J., Mix, A. C., Abram, N. J., Austermann, J., Brovkin, V., Capron, E., Colombaroli, D., Daniau, A.-L., Dyez, K. A., Felis, T., Finkelstein, S. A., Jaccard, S. L., McClymont, E. L., Rovere, A., Sutter, J., Wolff, E. W., Affolter, S., Bakker, P., Ballesteros-Cánovas, J. A., Barbante, C., Caley, T., Carlson, A. E., Churakova, O., Cortese, G., Cumming, B. F., Davis, B. A. S., de Vernal, A., Emile-Geay, J., Fritz, S. C., Gierz, P., Gottschalk, J., Holloway, M. D., Joos, F., Kucera, M., Loutre, M.-F., Lunt, D. J., Marcisz, K., Marlon, J. R., Martinez, P., Masson-Delmotte, V., Nehrbass-Ahles, C., Otto-Bliesner, B. L., Raible, C. C., Risebrobakken, B., Sánchez Goñi, M. F., Arrigo, J. S., Sarnthein, M., Sjolte, J., Stocker, T. F., Velasquez Alvárez, P. A., Tinner, W., Valdes, P. J., Vogel, H., Wanner, H., Yan, Q., Yu, Z., Ziegler, M., and Zhou, L.: Palaeoclimate constraints on the impact of 2 ∘C anthropogenic warming and beyond, Nat. Geosci., 11, 474–485, https://doi.org/10.1038/s41561-018-0146-0, 2018.
Fischer, H., Traufetter, F., Oerter, H., Weller, R., and Miller, H.: Prevalence of the Antarctic Circumpolar Wave over the last two millennia recorded in Dronning Maud Land ice, Geophys. Res. Lett., 31, L08202, https://doi.org/10.1029/2003GL019186, 2004.
Fogt, R. L., Sleinkofer, A. M., Raphael, M. N., and Handcock, M. S.: A regime shift in seasonal toal Antarctic sea ice extent in the twentieth century, Nat. Clim. Change, 12, 54–62, 2022.
Fraser, W. R., Pitman, R. L., and Ainley, D. G.: Seabird and fur seal responses to vertically migrating winter krill swarms in Antarctica, Pol. Biol., 10, 37–41, 1989.
Frey, M. M., Norris, S. J., Brooks, I. M., Anderson, P. S., Nishimura, K., Yang, X., Jones, A. E., Nerentorp Mastromonaco, M. G., Jones, D. H., and Wolff, E. W.: First direct observation of sea salt aerosol production from blowing snow above sea ice, Atmos. Chem. Phys., 20, 2549–2578, https://doi.org/10.5194/acp-20-2549-2020, 2020.
Gagné, M. È., Gillett, N. P., and Fyfe, J. C.: Observed and simulated changes in Antarctic sea ice extent over the past 50 years, Geophys. Res. Lett., 42, 90–95, 2015.
Galbraith, E. D. and Skinner, L. C.: The Biological Pump during the Last Glacial Maximum, Ann. Rev. Mar. Sci., 12, 559–586, 2020.
Garrison, D. L., Sullivan, C. W., and Ackley, S. F.: Sea Ice Microbial Communities in Antarctica, BioScience, 36, 243–250, 1986.
Gersonde, R., Abelmann, A., Brathauer, U., Becquey, S., Bianchi, C., Cortese, G., Grobe, H., Kuhn, G., Niebler, H. S., Segl, M., Sieger, R., Zielinski, U., and Futterer, D. K.: Last glacial sea surface temperatures and sea-ice extent in the Southern Ocean (Atlantic-Indian sector): A multiproxy approach, Paleoceanography, 18, 1061, https://doi.org/10.1029/2002PA000809, 2003.
Gersonde, R., Crosta, X., Abelmann, A., and Armand, L.: Sea-surface temperature and sea ice distribution of the Southern Ocean at the EPILOG Last Glacial Maximum – A circum-Antarctic view based on siliceous microfossil records, Quaternary Sci. Rev., 24 869–896, 2005.
Gersonde, R. and Zielinski, U.: The reconstruction of late Quaternary Antarctic sea-ice distribution – The use of diatoms as a proxy for sea-ice, Palaeogeogr. Palaeoclim. Palaeoecol., 162, 263–286, 2000.
Ghadi, P., Nair, A., Crosta, X., Mohan, R., Manoj, M. C., and Meloth, T.: Antarctic sea-ice and palaeoproductivity variation over the last 156,000 years in the Indian sector of Southern Ocean, Mar. Micropaleontol., 160, 101894, https://doi.org/10.1016/j.marmicro.2020.101894, 2020.
Gildor, H. and Tziperman, E.: Sea ice as the glacial cycle's climatic switch: Role of seasonal and orbital forcing, Paleoceanography, 15, 605–615, 2000.
Giordano, M. R., Kalnajs, L. E., Goetz, J. D., Avery, A. M., Katz, E., May, N. W., Leemon, A., Mattson, C., Pratt, K. A., and DeCarlo, P. F.: The importance of blowing snow to halogen-containing aerosol in coastal Antarctica: influence of source region versus wind speed, Atmos. Chem. Phys., 18, 16689–16711, https://doi.org/10.5194/acp-18-16689-2018, 2018.
Goosse, H. and Zunz, V.: Decadal trends in the Antarctic sea ice extent ultimately controlled by ice–ocean feedback, The Cryosphere, 8, 453–470, https://doi.org/10.5194/tc-8-453-2014, 2014.
Gordon, A. L.: Seasonality of Southern Ocean sea ice, J. Geophys.l Res., 86, 4193–4197, 1981.
Green, R. A., Menviel, L., Meissner, K. J., Crosta, X., Chandan, D., Lohmann, G., Peltier, W. R., Shi, X., and Zhu, J.: Evaluating seasonal sea-ice cover over the Southern Ocean at the Last Glacial Maximum, Clim. Past, 18, 845–862, https://doi.org/10.5194/cp-18-845-2022, 2022.
Greene, C. A., Young, D. A., Gwyther, D. E., Galton-Fenzi, B. K., and Blankenship, D. D.: Seasonal dynamics of Totten Ice Shelf controlled by sea ice buttressing, The Cryosphere, 12, 2869–2882, https://doi.org/10.5194/tc-12-2869-2018, 2018.
Hall, A.: The Role of Surface Albedo Feedback in Climate, J. Climate, 17, 1550–1568, 2004.
Hall, A. and Visbeck, M.: Synchronous variability in the Southern Hemisphere atmosphere, sea ice, and ocean resulting from the Annular Mode, J. Climate, 15, 3043–3057, 2002.
Hao, G., Shen, H., Sun, Y., and Li, C.: Rapid decrease in Antarctic sea ice in recent years, Acta Oceanologica Sinica, 40, 119–128, https://doi.org/10.1007/s13131-021-1762-x, 2021.
Hasle, G. R.: An analysis of the Phytoplankton of the Pacific Southern Ocean: abundance, composition, and distribution during the Brattegg Expedition, 1947–1948, Hvalradets Skrifter, 52, 1–168, 1969.
Hays, J. D., Lozano, J. A., Shackleton, N., and Irving, G.: Reconstruction of the Atlantic and western Indian ocean sectors of the 18,000 B. P. Antarctic Ocean, Geol. Soc. Am. Memoirs, 145, 337–372, 1976.
Heroy, D. C., Sjunneskog, C., and Anderson, J. B.: Holocene climate change in the Bransfield Basin, Antarctic Peninsula: Evidence from sediment and diatom analysis, Ant. Sci., 20, 69–87, 2008.
Hewitt, H. T., Roberts, M., Mathiot, P., Biastoch, A., Blockley, E., Chassignet, E. P., Fox-Kemper, B., Hyder, P., Marshall, D. P., Popova, E., Treguier, A.-M., Zanna, L., Yool, A., Yu, Y., Beadling, R., Bell, M., Kuhlbrodt, T., Arsouze, T., Bellucci, A., Castruccio, F., Gan, B., Putrasahan, D., Roberts, C. D., Van Roekel, L., and Zhang, Q.: Resolving and Parameterising the Ocean Mesoscale in Earth System Models, Curr. Clim. Change Rep., 6, 137–152, 2020.
Hobbs, W. R., Bindoff, N. L., and Raphael, M. N.: New perspectives on observed and simulated Antarctic sea ice extent trends using optimal fingerprinting techniques, J. Climate, 28, 1543–1560, 2014.
Hobbs, W. R., Massom, R., Stammerjohn, S., Reid, P., Williams, G., and Meier, W.: A review of recent changes in Southern Ocean sea ice, their drivers and forcings, Global Planet. Change, 143, 228–250, 2016.
Hobbs, W. R. and Raphael, M. N.: The Pacific zonal asymmetry and its influence on Southern Hemisphere sea ice variability, Ant. Sci., 22, 559–571, 2010.
Hoffman, J. S., Clark, P. U., Parnell, A. C., and Feng, H.: Regional and global sea-surface temperatures during the last interglaciation, Science, 355, 276–279, 2017.
Holland, M. M., Landrum, L., Raphael, M., and Stammerjohn, S.: Springtime winds drive Ross Sea ice variability and change in the following autumn, Nat. Commun., 8, 731, https://doi.org/10.1038/s41467-017-00820-0, 2017.
Holland, P. R.: The seasonality of Antarctic sea ice trends, Geophys. Res. Lett., 41, 4230–4237, 2014.
Holland, P. R. and Kwok, R.: Wind-driven trends in Antarctic sea-ice drift, Nat. Geosci., 5, 872–875, 2012.
Holloway, M. D., Sime, L. C., Allen, C. S., Hillenbrand, C.-D., Bunch, P., Wolff, E., and Valdes, P. J.: The spatial structure of the 128 ka Antarctic sea ice minimum, Geophys. Res. Lett., 44, 11129–11139, 2017.
Holloway, M. D., Sime, L. C., Singarayer, J. S., Tindall, J. C., Bunch, P., and Valdes, P. J.: Antarctic last interglacial isotope peak in response to sea ice retreat not ice-sheet collapse, Nat. Commun., 7, 12293, https://doi.org/10.1038/ncomms12293, 2016.
Holloway, M. D., Sime, L. C., Singarayer, J. S., Tindall, J. C., and Valdes, P. J.: Simulating the 128-ka Antarctic climate response to Northern Hemisphere ice sheet melting using the isotope-enabled HadCM3, Geophys. Res. Lett., 45, 11921–911929, 2018.
Hoppmann, M., Richter, M. E., Smith, I. J., Jendersie, S., Langhorne, P. J., Thomas, D. N., Dieckmann, G. S.: Platelet ice, the Southern Ocean's hidden ice: a review, Ann. Glaciol., 61, 341–368, 2020.
Huang, J., Jaeglé, L., and Shah, V.: Using CALIOP to constrain blowing snow emissions of sea salt aerosols over Arctic and Antarctic sea ice, Atmos. Chem. Phys., 18, 16253–16269, https://doi.org/10.5194/acp-18-16253-2018, 2018.
Hunke, E. C., Lipscomb, W. H., Turner, A. K., Jeffery, N., and Elliot, S.: CICE: The Los Alamos Sea Ice Model, documentation and software user's manual, version 5.1, Los Alamos Natl. Lab., Los Alamos, N. M. USA, 2015.
Hutson, W. H.: The Agulhas Current during the late Pleistocene: Analysis of modern faunal analogues, Science, 207, 64–66, 1980.
Iizuka, Y., Hondoh, T., and Fujii, Y.: Antarctic sea ice extent during the Holocene reconstructed from inland ice core evidence, J. Geophys. Res., 113, D15114, https://doi.org/10.1029/2007JD009326, 2008.
Imbrie, J. and Kipp, N.: A new micropaleontological method for quantitative paleoclimatology: Application to a late Pleistocene Caribbean core, in: Late Cenozoic Ages, edited by: Turekian, K. K., Yale University Press, New Haven, CT, 71–181, 1971.
Intergovernmental Panel on Climate Change (IPCC): The Physical Science Basis. Contribution of Working Group I to the Fifth Assessment Report of the Intergovernmental Panel on Climate Change, Cambridge University Press, Cambridge, United Kingdom and New York, NY, USA, 1535 pp., 2013.
Jeffries, M. O., Krouse, H. R., Hurst-Cushing, B., and Maksym, T.: Snow-ice accretion and snow-cover depletion on Antarctic first-year sea-ice floes, Ann. Glaciol., 33, 51–60, 2001.
Jena, B., Kumar, A., Ravichandran, M., and Kern, S.: Mechanism of sea-ice expansion in the Indian Ocean sector of Antarctica: Insights from satellite observation and model reanalysis, PloS one, 13, e0203222–e0203222, 2018.
Jones, J. M., Gille, S. T., Goosse, H., Abram, N. J., Canziani, P. O., Charman, D. J., Clem, K. R., Crosta, X., de Lavergne, C., Eisenman, I., England, M. H., Fogt, R. L., Frankcombe, L. M., Marshall, G. J., Masson-Delmotte, V., Morrison, A. K., Orsi, A. J., Raphael, M. N., Renwick, J. A., Schneider, D. P., Simpkins, G. R., Steig, E. J., Stenni, B., Swingedouw, D., and Vance, T. R.: Assessing recent trends in high-latitude Southern Hemisphere surface climate, Nat. Clim. Change, 6, 917–926, 2016.
Jones, J., Kohfeld, K. E., Bostock, H., Crosta, X., Liston, M., Dunbar, G., Chase, Z., Leventer, A., Anderson, H., and Jacobsen, G.: Sea ice changes in the southwest Pacific sector of the Southern Ocean during the last 140 000 years, Clim. Past, 18, 465–483, https://doi.org/10.5194/cp-18-465-2022, 2022.
Jourdain, B., Preunkert, S., Cerri, O., Castebrunet, H., Udisti, R., and Legrand, M.: Year-round record of size-segregated aerosol composition in central Antarctica (Concordia station): Implications for the degree of fractionation of sea-salt particles, J. Geophys. Res.-Atmos., 113, D14308, https://doi.org/10.1029/2007JD009584, 2008.
Kaczmarska, I., Barbrick, N. E., Ehrman, J. M., and Cant, G. P.: Eucampia Index as an indicator of the Late Pleistocene oscillations of the winter sea-ice extent at the ODP Leg 119 Site 745B at the Kerguelen Plateau, Hydrobiologia, 269/270, 103–112, 1993.
Kennedy, F., Martin, A., Castrisios, K., Cimoli, E., McMinn, A., and Ryan, K.G.: Rapid manipulation in irradiance induces oxidative free-radical release in a fast-ice algal community (McMurdo Sound, Antarctica), Front. Plant Sci., 11, 588005, https://doi.org/10.3389/fpls.2020.588005, 2020.
Kim, S., Yoo, K.-C., Lee, J. I., Khim, B.-K., Bak, Y.-S, Lee, M. K., Lee, J., Domack, E. W., Christ, A. J., and Yoon, H. I.: Holocene paleoceanography of Bigo Bay, west Antarctic Peninsula: Connections between surface water productivity and nutrient utilization and its implication for surface-deep water mass exchange, Quaternary Sci. Rev., 192, 59–70, 2018.
Kohfeld, K. E. and Chase, Z.: Temporal evolution of mechanisms controlling ocean carbon uptake during the last glacial cycle, Earth Planet. Sci. Lett., 472, 206–215, 2017.
Kohyama, T. and Hartmann, D. L.: Antarctic Sea Ice Response to Weather and Climate Modes of Variability, J. Climate, 29, 721–741, 2015.
Kusahara, K., Williams, G. D., Massom, R., Reid, P., and Hasumi, H.: Spatiotemporal dependence of Antarctic sea ice variability to dynamic and thermodynamic forcing: A coupled ocean–sea ice model study, Clim. Dynam., 52, 3791–3807, 2019.
Kwok, R. and Comiso, J. C.: Spatial patterns of variability in Antarctic surface temperature: Connections to the Southern Hemisphere Annular Mode and the Southern Oscillation, Geophys. Res. Lett., 29, 1705, https://doi.org/10.1029/2002GL015415, 2002.
Kwok, R., Comiso, J. C., Lee, T., and Holland, P. R.: Linked trends in the South Pacific sea ice edge and Southern Oscillation Index, Geophys. Res. Lett., 43, 10295–210302, 2016.
Labrousse, S., Sallée, J.-B., Fraser, A. D., Massom, R. A., Reid, P., Sumner, M., Guinet, C., Harcourt, R., McMahon, C., Bailleul, F., Hindell, M. A., and Charrassin, J.-B.: Under the sea ice: Exploring the relationship between sea ice and the foraging behaviour of southern elephant seals in East Antarctica, Prog. Oceanogr., 156, 17–40, 2017.
Labrousse, S., Williams, G., Tamura, T., Bestley, S., Sallée, J.-B., Fraser, A. D., Sumner, M., Roquet, F., Heerah, K., Picard, B., Guinet, C., Harcourt, R., McMahon, C., Hindell, M. A., and Charrassin, J.-B.: Coastal polynyas: Winter oases for subadult southern elephant seals in East Antarctica, Sci. Rep., 8, 3183, https://doi.org/10.1038/s41598-018-21388-9, 2018.
Lafond, A., Leblanc, K., Legras, J., Cornet, V., and Quéguiner, B.: The structure of diatom communities constrains biogeochemical properties in surface waters of the Southern Ocean (Kerguelen Plateau), J. Mar. Syst., 212, 103458, https://doi.org/10.1016/j.jmarsys.2020.103458, 2020.
Lamping, N., Müller, J., Hefter, J., Mollenhauer, G., Haas, C., Shi, X., Vorrath, M.-E., Lohmann, G., and Hillenbrand, C.-D.: Evaluation of lipid biomarkers as proxies for sea ice and ocean temperatures along the Antarctic continental margin, Clim. Past, 17, 2305–2326, https://doi.org/10.5194/cp-17-2305-2021, 2021.
Lamping, N., Müller, J., Esper, O., Hillenbrand, C.-D., Smith, J. A., and Kuhn, G.: Highly branched isoprenoids reveal onset of deglaciation followed by dynamic sea-ice conditions in the western Amundsen Sea, Antarctica, Quaternary Sci. Rev., 228, 106103, https://doi.org/10.1016/j.quascirev.2019.106103, 2020.
Landrum, L., Holland, M. M., Schneider, D. P., and Hunke, E.: Antarctic sea ice climatology, variability, and late twentieth-century change in CCSM4, J. Climate, 25, 4817–4838, 2012.
Langhorne, P. J., Hughes, K. G., Gough, A. J., Smith, I. J., Williams, M. J. M., Robinson, N. J., Stevens, C. L., Rack, W., Price, D., Leonard, G. H., Mahoney, A. R., Haas, C., and Haskell, T. G.: Observed platelet ice distributions in Antarctic sea ice: An index for ocean-ice shelf heat flux, Geophys. Res. Lett., 42, 5442–5451, 2015.
Lannuzel, D., Schoemann, V., de Jong, J., Pasquer, B., van der Merwe, P., Masson, F., Tison, J.-L., and Bowie, A.: Distribution of dissolved iron in Antarctic sea ice: Spatial, seasonal, and inter-annual variability, J. Geophys. Res., 115, G03022, https://doi.org/10.1029/2009JG001031, 2010.
Lawler, K.-A., Cortese, G., Civel-Mazens, M., Bostock, H., Crosta, X., Leventer, A., Lowe, V., Rogers, J., and Armand, L. K.: The Southern Ocean Radiolarian (SO-RAD) dataset: a new compilation of modern radiolarian census data, Earth Syst. Sci. Data, 13, 5441–5453, https://doi.org/10.5194/essd-13-5441-2021, 2021.
Lecomte, O., Goosse, H., Fichefet, T., de Lavergne, C., Barthélemy, A.., and Zunz, V.: Vertical ocean heat redistribution sustaining sea-ice concentration trends in the Ross Sea, Nat. Commun., 8, 258, https://doi.org/10.1038/s41467-017-00347-4, 2017.
Leventer, A.: The fate of sea ice diatoms and their use as paleoenvironmental indicators, in: Antarctic Sea Ice: Biological Processes, edited by: Lizotte, M. P. and Arrigo, K. R., American Geophysical Union, Washington, DC, USA, vol. 73, 121–137, 1998.
Leventer, A.: Modern distribution of diatoms in sediments from the George V Coast, Antarctica, Mar. Micropaleontol., 19, 315–332, 1992.
Leventer, A., Domack, E. W., Ishman, S. E., Brachfeld, S., McClennen, C. E., and Manley, P.: Productivity cycles of 200–300 years in the Antarctic Peninsula region: Understanding linkages among the sun, atmosphere, oceans, sea ice, and biota, GSA Bulletin, 108, 1626–1644, 1996.
Leventer, A., Dunbar, R. B., and DeMaster, D. J.: Diatom Evidence for Late Holocene Climatic Events in Granite Harbor, Antarctica, Paleoceanography, 8, 373–386, 1993.
Levine, J. G., Yang, X., Jones, A. E., and Wolff, E. W.: Sea salt as an ice core proxy for past sea ice extent: A process-based model study, J. Geophys. Res.-Atmos., 119, 5737–5756, 2014.
Lhardy, F., Bouttes, N., Roche, D. M., Crosta, X., Waelbroeck, C., and Paillard, D.: Impact of Southern Ocean surface conditions on deep ocean circulation during the LGM: a model analysis, Clim. Past, 17, 1139–1159, https://doi.org/10.5194/cp-17-1139-2021, 2021.
Li, S., Huang, G., Li, X., Liu, J., and Fan, G.: An assessment of the Antarctic sea ice mass budget simulation in CMIP6 historical experiment, Front. Earth Sci., 9, 649743,, https://doi.org/10.3389/feart.2021.649743, 2021.
Li, Q., Xiao, W., Wang, R., and Chen, Z.: Diatom based reconstruction of climate evolution through the Last Glacial Maximum to Holocene in the Cosmonaut Sea, East Antarctica, Deep-Sea Res. II, 194, 104960, 2021.
Liu, J., Martinson, D. G., Yuan, X., and Rind, D.: Evaluating Antarctic sea ice variability and its teleconnections in global climate models, Int. J. Climatol., 22, 885–900, 2002.
Loeb, V., Siegel, V., Holm-Hansen, O., Hewitt, R., Fraser, W., Trivelpiece, W., and Trivelpiece, S.: Effect of sea-ice extent and krill or salp dominance on the Antarctic food web, Nature, 387, 897–900, 1997.
Lowe, V., Cortese, G., Lawler, L.-A., Civel-Mazens, M., and Bostock, H. C.: Ecoregionalisation of the Southern Ocean using radiolarians, Front. Mar. Sci., 9, 829676, https://doi.org/10.3389/fmars.2022.829676, 2022.
Lund, D. C., Chase, Z., Kohfeld, K. E., and Wilson, E. A.: Tracking Southern Ocean sea ice extent with Winter Water: A new method based on the oxygen isotopic signature of foraminifera, Paleoceanogr. Paleoclimatol., 36, e2020PA004095, https://doi.org/10.1029/2020PA004095, 2021.
Mahowald, N. M., Lamarque, J. F., Tie, X. X., and Wolff, E.: Sea-salt aerosol response to climate change: Last Glacial Maximum, preindustrial, and doubled carbon dioxide climates, J. Geophys. Res.-Atmos., 111, D05303, https://doi.org/10.1029/2005JD006459, 2006.
Maksym, T.: Arctic and Antarctic Sea Ice Change: Contrasts, Commonalities, and Causes, Annu. Rev. Mar. Sci., 11, 187–213, 2019.
Maksym, T., Stammerjohn, S. E., Ackley, S., and Massom, R.: Antarctic Sea Ice – A Polar Opposite?, Oceanography, 25, 140–151, 2012.
Malmierca-Vallet, I., Sime, L. C., Tindall, J. C., Capron, E., Valdes, P. J., and Vinther, B. M.: Simulating the Last Interglacial Greenland stable water isotope peak: The role of Arctic sea ice changes, Quaternary Sci. Rev., 198, 1–14, 2018.
Marcott, S. A., Bauska, T. K., Buizert, C., Steig, E. J., Rosen, J. L., Cuffrey, K. M., Fudge, T. J., Severinghaus, J. P., Ahn, J., Kalk, M.L., McConnell, J. R., Sowers, T., Taylor, K. C., White, J. W. C., and Brook, E. J.: Centennial-scale changes in the global carbon cycle during the last deglaciation, Nature, 514, 616–619, 2014.
MARGO Project Members: Constraints on the magnitude and patterns of ocean cooling at the Last Glacial Maximum, Nat. Geosci., 2, 127–132, 2009.
Markle, B. R., Steig, E. J., Roe, G. H., Winckler, G., and McConnell, J. R.: Concomitant variability in high-latitude aerosols, water isotopes and the hydrologic cycle, Nat. Geosci., 11, 853–859, 2018.
Marzocchi, A. and Jansen, M. F.: Connecting Antarctic sea ice to deep-ocean circulation in modern and glacial climate simulations, Geophys. Res. Lett., 44, 6286–6295, 2017.
Marzocchi, A. and Jansen, M. F.: Global cooling linked to increased glacial carbon storage via changes in Antarctic sea ice, Nat. Geosci., 12, 1001–1005, 2019.
Massé, G., Belt, S. T., Crosta, X., Schmidt, S., Snape, I., Thomas, D. N., and Rowland, S. J.: Highly branched isoprenoids as proxies for variable sea ice conditions in the Southern Ocean, Antarc. Sci., 23, 487–498, 2011.
Massom, R. A., Eicken, H., Hass, C., Jeffries, M. O., Drinkwater, M. R., Sturm, M., Worby, A. P., Wu, X., Lytle, V. I., Ushio, S., Morris, K., Reid, P. A., Warren, S. G., and Allison, I.: Snow on Antarctic sea ice, Rev. Geophys., 39, 413–445, 2001.
Massom, R. A., Harris, P. T., Michael, K. J., and Potter, M. J.: The distribution and formative processes of latent-heat polynyas in East Antarctica, Ann. Glaciol., 27, 420–426, 1998.
Massom, R. A., Scambos, T. A., Bennetts, L. G., Reid, P., Squire, V. A., and Stammerjohn, S. E.: Antarctic ice shelf disintegration triggered by sea ice loss and ocean swell, Nature, 558, 383–389, 2018.
Massom, R. A. and Stammerjohn, S. E.: Antarctic sea ice change and variability – Physical and ecological implications, Polar Sci., 4, 149–186, 2010.
Masson-Delmotte, V., Buiron, D., Ekaykin, A., Frezzotti, M., Gallée, H., Jouzel, J., Krinner, G., Landais, A., Motoyama, H., Oerter, H., Pol, K., Pollard, D., Ritz, C., Schlosser, E., Sime, L. C., Sodemann, H., Stenni, B., Uemura, R., and Vimeux, F.: A comparison of the present and last interglacial periods in six Antarctic ice cores, Clim. Past, 7, 397–423, https://doi.org/10.5194/cp-7-397-2011, 2011.
Matear, R. J., O'Kane, T. J., Risbey, J. S., and Chamberlain, M.: Sources of heterogeneous variability and trends in Antarctic sea-ice, Nat. Commun., 6, 8656, https://doi.org/10.1038/ncomms9656, 2015.
McClymont, E. L., Bentley, M. J., Hodgson, D. A., Spencer-Jones, C. L., Wardley, T., West, M. D., Croudace, I. W., Berg, S., Gröcke, D. R., Kuhn, G., Jamieson, S. S. R., Sime, L., and Phillips, R. A.: Summer sea-ice variability on the Antarctic margin during the last glacial period reconstructed from snow petrel (Pagodroma nivea) stomach-oil deposits, Clim. Past, 18, 381–403, https://doi.org/10.5194/cp-18-381-2022, 2022.
Meehl, G. A., Arblaster, J. M., Bitz, C. M., Chung, C. T. Y., and Teng, H.: Antarctic sea-ice expansion between 2000 and 2014 driven by tropical Pacific decadal climate variability, Nat. Geosci., 9, 590–595, 2016.
Menviel, L., Joos, F., and Ritz, S. P.: Simulating atmospheric CO2, 13C and the marine carbon cycle during the Last Glacial-Interglacial cycle: possible role for a deepening of the mean remineralization depth and an increase in the oceanic nutrient inventory, Quaternary Sci. Rev., 56, 46–68, 2012.
Menviel, L., Spence, P., and England, M. H.: Contribution of enhanced Antarctic Bottom Water formation to Antarctic warm events and millennial-scale atmospheric CO2 increase, Earth Planet. Sci. Lett., 413, 37–50, 2015.
Menviel, L., Spence, P., Yu, J., Chamberlain, M. A., Matear, R. J., Meissner, K. J., and England, M. H.: Southern Hemisphere westerlies as a driver of the early deglacial atmospheric CO2 rise, Nat. Commun., 9, 2503, https://doi.org/10.1038/s41467-018-04876-4, 2018.
Mezgec, K., Stenni, B., Crosta, X., Masson-Delmotte, V., Baroni, C., Braida, M., Ciardini, V., Colizza, E., Melis, R., Salvatore, M. C., Severi, M., Scarchilli, C., Traversi, R., Udisti, R., and Frezzotti, M.: Holocene sea ice variability driven by wind and polynya efficiency in the Ross Sea, Nat. Commun., 8, 1334, https://doi.org/10.1038/s41467-017-01455-x, 2017.
Mix, A. C., Bard, E., and Schneider, R.: Environmental processes of the ice age: land, oceans, glaciers (EPILOG), Quaternary Sci. Rev., 20, 627–658, https://doi.org/10.1016/S0277-3791(00)00145-1, 2001.
Mohrmann, M., Heuzé, C., and Swart, S.: Southern Ocean polynyas in CMIP6 models, The Cryosphere, 15, 4281–4313, https://doi.org/10.5194/tc-15-4281-2021, 2021.
Nair, A., Mohan, R., Crosta, X., Manoj, M. C., Thamban, M., and Marieu, V.: Southern Ocean sea ice and frontal changes during the Lçte Quaternary and their linkages to Asian summer monsoon, Quaternary Sci. Rev., 213, 93–104, 2019.
Neori, A. and Holm-Hansen, O.: Effect of temperature on rate of photosynthesis in Antarctic phytoplankton, Polar Biol., 1, 33–38, 1982.
Nicholls, K. W., Østerhus, S., Makinson, K., Gammelsrød, T., and Fahrbach, E.: Ice-ocean processes over the continental shelf of the southern Weddell Sea, Antarctica: A review, Rev. Geophys., 47, RG3003, https://doi.org/10.1029/2007RG000250, 2009.
Nicolas, J. P., Vogelmann, A. M., Scott, R. C., Wilson, A. B., Cadeddu, M. P., Bromwich, D. H., Verlinde, J., Lubin, D., Russell, L. M., Jenkinson, C., Powers, H. H., Ryczek, M., Stone, G., and Wille, J. D.: January 2016 extensive summer melt in West Antarctica favoured by strong El Niño, Nat. Commun., 8, 15799, https://doi.org/10.1038/ncomms15799, 2017.
Nielsen, S. H. H., Koc, N., and Crosta, X.: Holocene climate in the Atlantic sector of the southern ocean: controlled by insolation or oceanic circulation?, Geol. Soc. Am., 32, 317–320, 2004.
Nissen, C. and Vogt, M.: Factors controlling the competition between Phaeocystis and diatoms in the Southern Ocean and implications for carbon export fluxes, Biogeosciences, 18, 251–283, https://doi.org/10.5194/bg-18-251-2021, 2021.
Noone, D. and Simmonds, I.: Sea ice control of water isotope transport to Antarctica and implications for ice core interpretation, J. Geophys. Res., 109, D07105, https://doi.org/10.1029/2003JD004228, 2004.
Norkko, A., Thrush, S. F., Cummings, V. J., Gibbs, M. M., Andrew, N. L., Norkko, J., and Schwarz, A.-M.: Trophic structure of coastal Antarctic food webs associated with changes in sea ice and food supply, Ecology, 88, 2810–2820, 2007.
Ohshima, K. I., Fukamachi, Y., Williams, G. D., Nihashi, S., Roquet, F., Kitade, Y., Tamura, T., Hirano, D., Herraiz-Borreguero, L., Field, I., Hindell, M., Aoki, S., and Wakatsuchi, M.: Antarctic Bottom Water production by intense sea-ice formation in the Cape Darnley polynya, Nat. Geosci., 6, 235–240, 2013.
Olbers, D., Gouretsky, V., Seiß, G., and Schröter, J.: Hydrographic Atlas of the Southern Ocean, Alfred-Wegener-Institut, Bremerhaven, https://epic.awi.de/id/eprint/2325/ (last access: 29 July 2022), 1992.
Orsi, A. H., Whitworth III, T., and Nowlin Jr., W. D.: On the meridional extent and fronts of the Antarctic Circumpolar Current, Deep-Sea Res. I, 42, 641–673, 1995.
Otto-Bliesner, B. L., Brady, E. C., Zhao, A., Brierley, C. M., Axford, Y., Capron, E., Govin, A., Hoffman, J. S., Isaacs, E., Kageyama, M., Scussolini, P., Tzedakis, P. C., Williams, C. J. R., Wolff, E., Abe-Ouchi, A., Braconnot, P., Ramos Buarque, S., Cao, J., de Vernal, A., Guarino, M. V., Guo, C., LeGrande, A. N., Lohmann, G., Meissner, K. J., Menviel, L., Morozova, P. A., Nisancioglu, K. H., O'ishi, R., Salas y Mélia, D., Shi, X., Sicard, M., Sime, L., Stepanek, C., Tomas, R., Volodin, E., Yeung, N. K. H., Zhang, Q., Zhang, Z., and Zheng, W.: Large-scale features of Last Interglacial climate: results from evaluating the lig127k simulations for the Coupled Model Intercomparison Project (CMIP6)–Paleoclimate Modeling Intercomparison Project (PMIP4), Clim. Past, 17, 63–94, https://doi.org/10.5194/cp-17-63-2021, 2021.
Pant, V., Moher, J., and Seelanki, V.: Multi-decadal variations in the oceanic CO2 uptake and biogeochemical parameters over the northern and southern high latitudes, Polar Sci., 18, 102–112, 2018.
Parkinson, C. L.: A 40-y record reveals gradual Antarctic sea ice increases followed by decreases at rates far exceeding the rates seen in the Arctic, P. Natl. Acad. Sci. USA, 116, 14414–14423, 2019.
Parkinson, C. L. and Cavalieri, D. J.: Antarctic sea ice variability and trends, 1979–2010, The Cryosphere, 6, 871–880, https://doi.org/10.5194/tc-6-871-2012, 2012.
Parkinson, C. L. and DiGirolamo, N. E.: Sea ice extents continue to set new records: Arctic, Antarctic, and global results, Remote Sens. Environ., 267, 112753, 2021.
Peck, V. L., Allen, C. S., Kender, S., McClymont, E. L., and Hodgson, D. A.: Oceanographic variability on the West Antarctic Peninsula during the Holocene and the influence of upper circumpolar deep water, Quaternary Sci. Rev., 119, 54–65, 2015.
Pellichero, V., Sallée, J.-B., Chapman, C. C., and Downes, S. M.: The southern ocean meridional overturning in the sea-ice sector is driven by freshwater fluxes, Nat. Commun., 9, 1789, https://doi.org/10.1038/s41467-018-04101-2, 2018.
Petit, J. R. and Delmonte, B.: A model for large glacial–interglacial climate-induced changes in dust and sea salt concentrations in deep ice cores (central Antarctica): Palaeoclimatic implications and prospects for refining ice core chronologies, Tellus B, 61, 768–790, 2009.
Petrich, C. and Eicken, H.: Overview of sea ice growth and properties, in: Sea Ice, Third Edition, edited by: Thomas, D. N., Wiley-Blackwell, Oxford, UK, 1–41, ISBN 9781118778388, 2017.
Pike, J., Crosta, X., Maddison, E. J., Stickley, C. E., Denis, D., Barbara, L., and Renssen, H.: Observations on the relationship between the Antarctic coastal diatoms Thalassiosira antarctica Comber and Porosira glacialis (Grunow) Jørgensen and sea ice concentrations during the late Quaternary, Mar. Micropaleontol., 73, 14–25, 2009.
Pike, J., Swann, G. E. A., Leng, M. J., and Snelling, A. M.: Glacial discharge along the west Antarctic Peninsula during the Holocene, Nat. Geosci., 6, 199–202, 2013.
Polvani, L. M. and Smith, K. L.: Can natural variability explain observed Antarctic sea ice trends? New modeling evidence from CMIP5, Geophys. Res. Lett., 40, 3195–3199, 2013.
Prebble, J. G., Crouch, E. M., Carter, L., Cortese, G., Bostock, H. C., and Neil, H.: An expanded modern dinoflagellate cyst dataset for the Southwest Pacific and Southern Hemisphere with environmental associations, Mar. Micropaleontol., 101, 33–48, 2013.
Purich, A., Cai, W., England, M. H., and Cowan, T.: Evidence for link between modelled trends in Antarctic sea ice and underestimated westerly wind changes, Nat. Commun., 7, 10409, https://doi.org/10.1038/ncomms10409, 2016.
Purkey, S. G., W. M. Smethie Jr., Gebbie, G., Gordon, A. L., Sonnerup, R. E., Warner, M. J., and Bullister, J. L.: A Synoptic View of the Ventilation and Circulation of Antarctic Bottom Water from Chlorofluorocarbons and Natural Tracers, Ann. Rev. Mar. Sci., 10, 503–527, 2018.
Rackow, T., Danilov, S., Goessling, H. F., Hellmer, H. H., Sein, D. V., Semmler, T., Sidorenko, D., and Jung, T.: Delayed Antarctic sea-ice decline in high-resolution climate change simulations, Nat. Commun., 13, 637, https://doi.org/10.1038/s41467-022-28259-y, 2022.
Ragueneau, O., Tréguer, P., Leynaert, A., Anderson, R. F., Brzezinski, M. A., DeMaster, D. J., Dugdale, R. C., Dymond, J., Fischer, G., François, R., Heinze, C., Maier-Reimer, E., Martin-Jézéquel, V., Nelson, D. M., and Quéguiner, B.: A review of the Si cycle in the modern ocean: recent progress and missing gaps in the application of biogenic opal as a paleoproductivity proxy, Global Planet. Change, 26, 317–365, 2000.
Rahaman, W., Thamban, M., and Laluraj, C.: Twentieth-century sea ice variability in the Weddell Sea and its effect on moisture transport: Evidence from a coastal East Antarctic ice core record, The Holocene, 26, 338–349, 2016.
Rankin, A. M., Wolff, E. W., and Martin, S.: Frost flowers: Implications for tropospheric chemistry and ice core interpretation, J. Geophys. Res.-Atmos., 107, 4683, https://doi.org/10.1029/2002JD002492, 2002.
Raphael, M. N.: The influence of atmospheric zonal wave three on Antarctic sea ice variability, J. Geophys. Res.-Atmos., 112, D12112, https://doi.org/10.1029/2006JD007852, 2007.
Reader, M. C. and McFarlane, N.: Sea-salt aerosol distribution during the Last Glacial Maximum and its implications for mineral dust, J. Geophys. Res.-Atmos., 108, 4253, https://doi.org/10.1029/2002JD002063, 2003.
Renssen, H., Goosse, H., Fichefet, T., Masson-Delmotte, V., and Koç, N.: Holocene climate evolution in the high-latitude Southern Hemisphere simulated by a coupled atmosphere-sea ice-ocean-vegetation model, The Holocene, 15, 951–964, 2005.
Rhodes, R. H., Kohfeld, K. E., Bostock, H., Crosta, X., Leventer, A., Meissner, K., and Esper, O.: Understanding past changes in sea ice in the Southern Ocean, PAGES Magazine, 27, 31, https://doi.org/10.22498/pages.27.1.31, 2019.
Rhodes, R. H., Yang, X., and Wolff, E. W.: Sea Ice Versus Storms: What Controls Sea Salt in Arctic Ice Cores?, Geophys. Res. Lett., 45, 5572–5580, 2018.
Riaux-Gobin, C., Dieckmann, G. S., Poulin, M., Neveux, J., Labrune, C., and Vétion, G.: Environmental conditions, particle flux and sympagic microalgal succession in spring before the sea-ice break-up in Adélie Land, East Antarctica, Pol. Res., 32, 19675, https://doi.org/10.3402/polar.v32i0.19675, 2013.
Riaux-Gobin, C. and Poulin, M.: Possible symbiosis of Berkeleya adeliensis Medlin, Synedropsis fragilis (Manguin) Hasle et al. and Nitzschia lecointei van Heurck (Bacillariophyta) associated with land-fast ice in Adelie Land, Ant. Diatom Res., 19, 265–274, 2004.
Rigual-Hernández, A. S., Trull, T. W., Gray, S. G., and Armand, L. K.: The fate of diatom valves in the Subantarctic and Polar Frontal Zones of the Southern Ocean: Sediment trap versus surface sediment assemblages, Palaeogeogr. Palaeoclimatol. Palaeoecol., 457, 129–143, 2016.
Rintoul, S. R.: The global influence of localized dynamics in the Southern Ocean, Nature, 558, 209–218, 2018.
Rintoul, S. R.: On the Origin and Influence of Adélie Land Bottom Water, in: Ocean, Ice, and Atmosphere: Interactions at the Antarctic Continental Margin, edited byL Jacobs, S. S. and Weiss, R. F., Antarctic Research Series, vol. 75, American Geophysical Union, Washington, DC, 151–171, 1998.
Roach, L. A., Bitz, C. M., Horvat, C., and Dean, S. M.: Advances in Modeling Interactions Between Sea Ice and Ocean Surface Waves, J. Adv. Model. Earth Syst., 11, 4167–4181, 2019.
Roach, L. A., Dörr, J., Holmes, C. R., Massonnet, F., Blockley, E. W., Notz, D., Rackow, T., Raphael, M. N., O'Farrell, S. P., Bailey, D. A., and Bitz, C. M.: Antarctic sea ice in CMIP6, Geophys. Res. Lett., 47, e2019GL086729, https://doi.org/10.1029/2019GL086729, 2020.
Roche, D. M., Crosta, X., and Renssen, H.: Evaluating Southern Ocean sea-ice for the Last Glacial Maximum and pre-industrial climates: PMIP-2 models and data evidence, Quaternary Sci. Rev., 56, 99–106, 2012.
Romero, O. E., Armand, L. K., Crosta, X., and Pichon, J. J.: The biogeography of major diatom taxa in Southern Ocean surface sediments: 3. Tropical/Subtropical species, Palaeogeogr. Palaeoclimatol. Palaeoecol., 223, 49–65, 2005.
Rontani, J.-F., Smik, L., Belt, S. T., Vaultier, F., Armbrecht, L., Leventer, A., and Armand, L. K.: Abiotic degradation of highly branched isoprenoid alkenes and other lipids in the water column off East Antarctica, Mar. Chem., 210, 34–47, 2019.
Rontani, J. F., Belt, S. T., Vaultier, F., Brown, T. A., and Massé, G.: Autoxidative and photooxidative reactivity of Highly Branched Isoprenoid (HBI) alkenes, Lipids, 49, 481–494, 2014.
Roscoe, H. K., Brooks, B., Jackson, A. V., Smith, M. H., Walker, S. J., Obbard, R. W., and Wolff, E. W.: Frost flowers in the laboratory: Growth, characteristics, aerosol, and the underlying sea ice, J. Geophys. Res.-Atmos., 116, D12301, https://doi.org/10.1029/2010JD015144, 2011.
Rossi, L., Sporta Caputi, S., Calizza, E., Careddu, G., Oliverio, M., Schiaparelli, S., and Constantini, M.L.: Antarctic food web architecture under varying dynamics of sea ice cover, Sci. Rep., 9, 12454, https://doi.org/10.1038/s41598-019-48245-7, 2019.
Röthlisberger, R., Bigler, M., Wolff, E. W., Joos, F., Monnin, E., Hutterli, M. A.: Ice core evidence for the extent of past atmospheric CO2 change due to iron fertilisation, Geophys. Res. Lett., 31, L16207, https://doi.org/10.1029/2004GL020338, 2004.
Röthlisberger, R., Crosta, X., Abram, N. J., Armand, L., and Wolff, E. W.: Potential and limitations of marine and ice core sea ice proxies: An example from the Indian Ocean sector, Quaternary Sci. Rev., 29, 296–302, 2010.
Rye, C. D., Marshall, J., Kelley, M., Russell, G., Nazarenko, L. S., Kostov, Y., Schmidt, G. A., and Hansen, J.: Antarctic glacial melt as a driver of recent Southern Ocean climate trends, Geophys. Res. Lett., 47, e2019GL086892, https://doi.org/10.1029/2019GL086892, 2020.
Rysgaard, S., Bendtsen, J., Delille, B., Dieckmann, G. S., Glud, R. N., Kennedy, H., Mortensen, J., Papadimitriou, S., Thomas, D. N., and Tison, J.-L.: Sea ice contribution to the air–sea CO2 exchange in the Arctic and Southern oceans, Tellus B, 63, 823–830, 2011.
Sabu, P., Subeesh, M. P., Sivakrishnan, K. K., and Anilkumar, N.: Causes and impacts of anomalous warming in the Prydz Bay, East Antarctica during austral summer 2016-17, Polar Sci., 30, 100660, https://doi.org/10.1016/j.polar.2021.100660, 2021.
Sarmiento, J. L., Gruber, N., Brzezinski, M. A., and Dunne, J. P.: High-latitude controls of thermocline nutrients and low latitude biological productivity, Nature, 427, 56–60, 2004.
Schönhardt, A., Begoin, M., Richter, A., Wittrock, F., Kaleschke, L., Gómez Martín, J. C., and Burrows, J. P.: Simultaneous satellite observations of IO and BrO over Antarctica, Atmos. Chem. Phys., 12, 6565–6580, https://doi.org/10.5194/acp-12-6565-2012, 2012.
Seguin, A. M., Norman, A.-L., and Barrie, L.: Evidence of sea ice source in aerosol sulfate loading and size distribution in the Canadian High Arctic from isotopic analysis, J. Geophys. Res.-Atmos., 119, 1087–1096, 2014.
Severi, M., Becagli, S., Caiazzo, L., Ciardini, V., Colizza, E., Giardi, F., Mezgec, K., Scarchilli, C., Stenni, B., Thomas, E. R., Traversi, R., and Udisti, R.: Sea salt sodium record from Talos Dome (East Antarctica) as a potential proxy of the Antarctic past sea ice extent, Chemosphere, 177, 266–274, 2017.
Shemesh, A., Hodell, D., Crosta, X., Kanfoush, S., Charles, C., and Guilderson, T.: Sequence of events during the last deglaciation in Southern Ocean sediments and Antarctic ice cores, Paleoceanography, 17, 1056, https://doi.org/10.1029/2000PA000599, 2002.
Shevenell, A., Ingalls, A. E., Domack, E. W., and Kelly, C.: Holocene Southern Ocean temperature variability west of the Antarctic Peninsula, Nature, 470, 250–254, 2011.
Shin, S. I., Liu, Z., Otto-Bliesner, B. L., Brady, E. C., Kutzbach, J. E., and Vavrus, S.: Southern Ocean sea-ice control on the glacial North Atlantic thermohaline circulation, Geophys. Res. Lett., 30, 1096, https://doi.org/10.1029/2002GL015513, 2003.
Shu, Q., Song, Z., and Qiao, F.: Assessment of sea ice simulations in the CMIP5 models, The Cryosphere, 9, 399–409, https://doi.org/10.5194/tc-9-399-2015, 2015.
Shukla, S. K., Crosta, X., and Ikehara, M.: Sea surface temperatures in the Indian Sub-Antarctic Southern Ocean for the four interglacial periods, Geophys. Res. Lett., 48, e2020GL090994, https://doi.org/10.1029/2020GL090994, 2021.
Sigmond, M. and Fyfe, J. C.: Has the ozone hole contributed to increased Antarctic sea ice extent?, Geophys. Res. Lett., 37, L18502, https://doi.org/10.1029/2010GL044301, 2010.
Sime, L. C., Hopcroft, P. O., and Rhodes, R. H.: Impact of abrupt sea ice loss on Greenland water isotopes during the last glacial period, P. Natl. Acad. Sci. USA, 116, 4099–4104, 2019.
Simmonds, I.: Comparing and contrasting the behaviour of Arctic and Antarctic sea ice over the 35 year period 1979-2013, Ann. Glaciol., 56, 18–28, 2015.
Simpkins, G. R., Ciasto, L. M., Thompson, D. W. J., and England, M. H.: Seasonal Relationships between Large-Scale Climate Variability and Antarctic Sea Ice Concentration, J. Climate, 25, 5451–5469, 2012.
Singh, H. K. A., Landrum, L., Holland, M. M., Bailey, D. A., and DuVivier, A. K.: An overview of Antarctic sea ice in the Community Earth System Model version 2, part I: Analysis of the seasonal cycle in the context of sea ice thermodynamics and coupled atmosphere-ocean-ice processes, J. Adv. Model. Earth Syst., 12, e2020MS002143, https://doi.org/10.1029/2020MS002143, 2020.
Sinninghe Damsté, J. S., Rijpstra, W. I. C., Coolen, M. J. L., Schouten, S., and Volkman, J. K.: Rapid sulfurisation of highly branched isoprenoid (HBI) alkenes in sulfidic Holocene sediments from Ellis Fjord, Antarctica, Org. Geochem., 38, 128–139, 2007.
Sjunneskog, C. and Taylor, F.: Postglacial marine diatom record of the Palmer Deep, Antarctic Peninsula (ODP Leg 178, Site 1098) 1. Total diatom abundance, Paleoceanography, 17, 10, https://doi.org/10.1029/2000PA000563, 2002.
Smik, L., Belt, S. T., Lieser, J. L., Armand, L. K., and Leventer, A.: Distributions of highly branched isoprenoid alkenes and other algal lipids in surface waters from East Antarctica: Further insights for biomarker-based paleo sea-ice reconstruction, Org. Geochem., 95, 71–80, 2016.
Spolaor, A., Vallelonga, P., Plane, J. M. C., Kehrwald, N., Gabrieli, J., Varin, C., Turetta, C., Cozzi, G., Kumar, R., Boutron, C., and Barbante, C.: Halogen species record Antarctic sea ice extent over glacial–interglacial periods, Atmos. Chem. Phys., 13, 6623–6635, https://doi.org/10.5194/acp-13-6623-2013, 2013.
Stein, K., Timmermann, A., Kwon, E. Y., and Friedrich, T.: Timing and magnitude of Southern Ocean sea ice/carbon cycle feedbacks, P. Natl. Acad. Sci. USA, 117. 4498–4504, 2020.
Stuecker, M. F., Bitz, C. M., and Armour, K. C.: Conditions leading to the unprecedented low Antarctic sea ice extent during the 2016 austral spring season, Geophys. Res. Lett., 44, 9008–9019, 2017.
Sturm, M. and Massom, R. A.: Snow in the sea ice system: friend or foe?, in: Sea Ice, 3rd Edition, edited by: Thomas, D. N., Wiley-Blackwell, Oxford, UK, 65–109, 2017.
Swingedouw, D., Fichefet, T., Huybrechts, P., Goosse, H., Driesschaert, E., and Loutre, M.-F.: Antarctic ice-sheet melting provides negative feedbacks on future climate warming, Geophys. Res. Lett., 35, L17705, https://doi.org/10.1029/2008GL034410, 2008.
Takao, S., Nakaoka, S.-I., Hashihama, F., Shimada, K., Yoshikawa-Inoue, H., Hirawake, T., Kanda, J., Hashida, G., and Suzuki, K.: Effects of phytoplankton community composition and productivity on sea surface pCO2 variations in the Southern Ocean, Deep Sea Res. Part I, 160, 103263, 2020.
Tamsitt, V., Drake, H. F., Morrison, A. K., Talley, L. D., Dufour, C. O., Gray, A. R., Griffies, S. M., Mazloff, M. R., Sarmiento, J. L., Wang, J., and Weijer, W.: Spiraling pathways of global deep waters to the surface of the Southern Ocean, Nat. Commun., 8, 172, 2017.
Taylor, F. and Sjunneskog, C.: Postglacial marine diatom record of the Palmer Deep, Antarctic Peninsula (ODP Leg 178, Site 1098) 2. Diatom assemblages, Paleoceanography, 17, 8001, https://doi.org/10.1029/2000PA000564, 2002.
Taylor, F., Whitehead, J., and Domack, E.: Holocene paleoclimate change in the Antarctic Peninsula: evidence from the diatom, sedimentary and geochemical record, Mar. Micropaleontol., 41, 25–43, 2001.
Thomas, D. N. and Dieckmann, G. S.: Antarctic sea ice: A habitat for extremophile, Science, 295, 641–644, https://doi.org/10.1126/science.1063391, 2022.
Thomas, E. R., Allen, C. S., Etourneau, J., King, A. C. F., Severi, M., Winton, V. H. L., Mueller, J., Crosta, X., and Peck, V. L.: Antarctic sea ice proxies from Marine and ice core archives suitable for reconstructing sea ice over the past 2000 Years, Geosciences, 9, 506, https://doi.org/10.3390/geosciences9120506, 2019.
Totten, R. L., Reuel Fonseca, A. N., Smith Wellner, J., Munoz, Y. P., Anderson, J. B., Tobin, T. S., and Lehrmann, A. A.: Oceanographic and climatic influences on Trooz Glacier, Antarctica, during the Holocene, Quaternary Sci. Rev., 276, 107279, https://doi.org/10.1016/j.quascirev.2021.107279, 2022.
Turner, J., Hosking, J. S., Marshall, G. J., Phillips, T., and Bracegirdle, T. J.: Antarctic sea ice increase consistent with intrinsic variability of the Amundsen Sea Low, Clim. Dynam., 46, 2391–2402, 2016.
Turner, J., Hosking, J. S., Phillips, T., and Marshall, G. J.: Temporal and spatial evolution of the Antarctic sea ice prior to the September 2012 record maximum extent, Geophys. Res. Lett., 40, 5894–5898, 2013.
Turner, J., Phillips, T., Marshall, G. J., Hosking, J. S., Pope, J. O., Bracegirdle, T. J., and Deb, P.: Unprecedented springtime retreat of Antarctic sea ice in 2016, Geophys. Res. Lett., 44, 6868–6875, 2017.
Vallelonga, P., Maffezzoli, N., Moy, A. D., Curran, M. A. J., Vance, T. R., Edwards, R., Hughes, G., Barker, E., Spreen, G., Saiz-Lopez, A., Corella, J. P., Cuevas, C. A., and Spolaor, A.: Sea-ice-related halogen enrichment at Law Dome, coastal East Antarctica, Clim. Past, 13, 171–184, https://doi.org/10.5194/cp-13-171-2017, 2017.
Vallelonga, P., Maffezzoli, N., Saiz-Lopez, A., Scoto, F., Kjaer, H.A., and Spolar, A.: Sea-ice reconstructions from bromine and iodine in ice cores, Quaternary Sci. Rev., 269, 107133, 2021.
Vance, T. R., van Ommen, T. D., Curran, M. A. J., Plummer, C. T., and Moy, A. D.: A millennial proxy record of ENSO and Eastern Australian rainfall from the Law Dome ice core, East Antarctica, J. Climate, 26, 710–725, 2013.
Vancoppenolle, M., Fichefet, T., and Goosse, H.: Simulating the mass balance and salinity of Arctic and Antarctic sea ice. 2. Importance of sea ice salinity variations, Ocean Modell., 27, 54–69, 2009.
Vancoppenolle, M., Meiners, K. M., Michel, C., Bopp, L., Brabant, F., Carnat, G., Delille, B., Lannuzel, D., Madec, G., Moreau, S., Tison, J.-L., and van der Merwe, P.: Role of sea ice in global biogeochemical cycles: Emerging views and challenges, Quaternary Sci. Rev., 79, 207–230, 2013.
Van Den Broeke, M., Reijmer, C., Van As, D., Van de Wal, R., and Oerlemans, J.: Seasonal cycles of Antarctic surface energy balance from automatic weather stations, Ann. Glaciol., 41, 131–139, 2005.
Van Leeuwe, M. A., Tedesco, L., Arrigo, K. R., Assmy, P., Campbell, K., Meiners, K. M., Rintala, J.-M., Selz, V., Thomas, D. N., and Stefels, J.: Microalgal community structure and primary production in Arctic and Antarctic sea ice: A synthesis, Elem. Sci. Anth., 6, https://doi.org/10.1525/elementa.267, 2018.
Veres, D., Bazin, L., Landais, A., Toyé Mahamadou Kele, H., Lemieux-Dudon, B., Parrenin, F., Martinerie, P., Blayo, E., Blunier, T., Capron, E., Chappellaz, J., Rasmussen, S. O., Severi, M., Svensson, A., Vinther, B., and Wolff, E. W.: The Antarctic ice core chronology (AICC2012): an optimized multi-parameter and multi-site dating approach for the last 120 thousand years, Clim. Past, 9, 1733–1748, https://doi.org/10.5194/cp-9-1733-2013, 2013.
Vernet, M., Geibert, W., Hoppema, M., Brown, P. J., Haas, C., Hellmer, H. H., Jokat, W., Jullion, L., Mazloff, M., Bakker, D. C. E., Brearley, J. A., Croot, P., Hattermann, T., Hauck, J., Hillenbrand, C.-D., Hoppe, C. J. M., Huhn, O., Koch, B. P., Lechtenfeld, O. J., Meredith, M. P., Naveira Garabato, A. C., Nöthig, E.-M., Peeken, I., Rutgers van der Loeff, M. M., Schmidtko, S., Schröder, M., Strass, V. H., Torres-Valdés, S., and Verdy, A.: The Weddell Gyre, Southern Ocean: Present Knowledge and Future Challenges, Rev. Geophys., 57, 623–708, 2019.
Von Quillfeldt, C. H.: The diatom Fragilariopsis cylindrus and its potential as an indicator species for cold water rather than for sea ice, Vie Milieu, 54, 137–143, 2004.
Vorrath, M.-E., Müller, J., Esper, O., Mollenhauer, G., Haas, C., Schefuß, E., and Fahl, K.: Highly branched isoprenoids for Southern Ocean sea ice reconstructions: a pilot study from the Western Antarctic Peninsula, Biogeosciences, 16, 2961–2981, https://doi.org/10.5194/bg-16-2961-2019, 2019.
Vorrath, M.-E., Müller, J., Rebolledo, L., Cárdenas, P., Shi, X., Esper, O., Opel, T., Geibert, W., Muñoz, P., Haas, C., Kuhn, G., Lange, C. B., Lohmann, G., and Mollenhauer, G.: Sea ice dynamics in the Bransfield Strait, Antarctic Peninsula, during the past 240 years: a multi-proxy intercomparison study, Clim. Past, 16, 2459–2483, https://doi.org/10.5194/cp-16-2459-2020, 2020.
Wadhams, P., Lange, M. A., and Ackley, S. F.: The ice thickness distribution across the Atlantic sector of the Antarctic Ocean in midwinter, J. Geophys. Res.-Oceans, 92, 14535–14552, 1987.
Wagenbach, D., Ducroz, F., Mulvaney, R., Keck, L., Minikin, A., Legrand, M., Hall, J. S., and Wolff, E. W.: Sea-salt aerosol in coastal Antarctic regions, J. Geophys. Res.-Atmos., 103, 10961–10974, 1998.
Wang, X. and Wu, Z.: Variability in Polar Sea Ice (1989–2018), IEEE Geosci. Remote Sens. Lett., 18, 1520–1524, 2021.
Weber, M. E., Bailey, I., Hemming, S. R., Martos, Y. M., Reilly, B. T., Ronge, T. A., Brachfeld, S., Williams, T., Raymo, M., Belt, S. T., Smik, L., Vogel, H., Peck, V. L., Armbrecht, L., Cage, A., Cardillo, F. G., Du, Z., Fauth, G., Fogwill, C. J., Garcia, M., Garnworthy, M., Glüder, A., Guitard, M., Gutjahr, M., Hernández-Almeida, I., Hoem, F. S., Hwang, J.-H., Iizuka, M., Kato, Y., Kenlee, B., OConnell, S., Pérez, L. F., Seki, O., Stevens, L., Tauxe, L., Tripathi, S., Warnock, J., and Zheng, X.: Antiphased dust deposition and productivity in the Antarctic Zone over te 1.5 million years, Nat. Commun., 13, 2044, https://doi.org/10.1038/s41467-022-29642-5, 2022.
Weeks, W. F. and Ackley, S. F.: The growth, structure, and properties of sea ice, CRREL Monograph, 81-1, 129 pp., 1982.
Weller, R., Traufetter, F., Fischer, H., Oerter, H., Piel, C., and Miller, H.: Postdepositional losses of methane sulfonate, nitrate, and chloride at the European Project for Ice Coring in Antarctica deep-drilling site in Dronning Maud Land, Antarctica, J. Geophys. Res.-Atmos., 109, D07301, https://doi.org/10.1029/2003JD004189, 2004.
White, W. B. and Peterson, R. G.: An Antarctic circumpolar wave in surface pressure, wind, temperature and sea-ice extent, Nature, 380, 699–702, 1996.
Whitehead, J. M. and McMinn, A.: Kerguelen Plateau Quaternary–late Pliocene palaeoenvironments: From diatom, silicoflagellate and sedimentological data, Palaeogeogr. Palaeoclimatol. Palaeoecol., 186, 335–368, 2002.
Whitehead, J. M., Wotherspoon, S., and Bohaty, S. M.: Minimal Antarctic sea ice during the Pliocene, Geology, 33, 137–140, 2005.
Wing, S. R., Leicther, J. J., Wing, L. C., Stokes, D., Genovese, S. J., McMullin, R. M., and Shatova, O. A.: Contribution of sea ice microbial production to Antarctic benthic communities is drvien by sea ice dynamics and composition of functional guilds, Global Change Biol., 24, 3642–3653, 2018.
Winski, D. A., Osterberg, E. C., Kreutz, K. J., Ferris, D. G., Cole-Dai, J., Thundercloud, Z., Huang, J., Alexander, B., Jaeglé, L., Kennedy, J. A., Larrick, C., Kahle, E. C., Steig, E. J., and Jones, T. R.: Seasonally resolved Holocene sea ice variability inferred from South Pole ice core chemistry, Geophys. Res. Lett., 48, e2020GL091602, https://doi.org/10.1029/2020GL091602, 2021.
Wolff, E. W., Barbante, C., Becagli, S., Bigler, M., Boutron, C. F., Castellano, E., de Angelis, M., Federer, U., Fischer, H., Fundel, F., Hansson, M., Hutterli, M., Jonsell, U., Karlin, T., Kaufmann, P., Lambert, F., Littot, G. C., Mulvaney, R., Röhlisberger, R., Ruth, U., Severi, M., Siggaard-Andersen, M. L., Sime, L. C., Steffensen, J. P., Stocker, T. F., Traversi, R., Twarloh, B., Udisti, R., Wagenbach, D., and Wegner, A.: Changes in environment over the last 800,000 years from chemical analysis of the EPICA Dome C ice core, Quaternary Sci. Rev., 29, 285–295, 2010.
Wolff, E. W., Fischer, H., Fundel, F., Ruth, U., Twarloh, B., Littot, G. C., Mulvaney, R., Röthlisberger, R., de Angelis, M., Boutron, C. F., Hansson, M., Jonsell, U., Hutterli, M. A., Lambert, F., Kaufmann, P., Stauffer, B., Stocker, T. F., Steffensen, J. P., Bigler, M., Siggaard-Andersen, M. L., Udisti, R., Becagli, S., Castellano, E., Severi, M., Wagenbach, D., Barbante, C., Gabrielli, P., and Gaspari, V.: Southern Ocean sea-ice extent, productivity and iron flux over the past eight glacial cycles, Nature, 440, 491–496, 2006.
Wolff, E.W., Rhodes, R.H., and Legrand, M.: Chapter 7 - Sea Salt in the Polar Regions, in: Advances in Atmospheric Chemistry, volume 3: Chemistry in the Cryosphere Part 1, edited by: Shepson, P. B. and Domine, F., World Scientific Publishing Co. Pte. Ltd., Singapore, 365–410 pp., 2021.
Worby, A. P., Geiger, C. A., Paget, M. J., van Woert, M. L., Ackley, S. F., and DeLiberty, T. L.: Thickness distribution of Antarctic sea ice, J. Geophys. Res., 113, C05S92, https://doi.org/10.1029/2007JC004254, 2008.
Wright, S. W. and van den Enden, R. L.: Phytoplankton community structure and stocks in the East Antarctic marginal ice zone (BROKE survey, January–March 1996) determined by CHEMTAX analysis of HPLC pigment signatures, Deep-Sea Res. II, 47, 2363–2400, 2000.
Wright, S. W., van den Enden, R. L., Pearce, I., Davidson, A. T., Scott, F. J., and Westwood, K. J.: Phytoplankton community structure and stocks in the Southern Ocean (30–80∘ E) determined by CHEMTAX analysis of HPLC pigment signatures, Deep-Sea Res. II, 57, 758–778, 2010.
Xiao, W. S., Esper, O., and Gersonde, R.: Last Glacial – Holocene climate variability in the Atlantic sector of the Southern Ocean, Quaternary Sci. Rev., 135, 115–137, 2016.
Yang, X., Frey, M. M., Rhodes, R. H., Norris, S. J., Brooks, I. M., Anderson, P. S., Nishimura, K., Jones, A. E., and Wolff, E. W.: Sea salt aerosol production via sublimating wind-blown saline snow particles over sea ice: parameterizations and relevant microphysical mechanisms, Atmos. Chem. Phys., 19, 8407–8424, https://doi.org/10.5194/acp-19-8407-2019, 2019.
Yang, X., Pyle, J. A., and Cox, R. A.: Sea salt aerosol production and bromine release: Role of snow on sea ice, Geophys. Res. Lett., 35, L16815, https://doi.org/10.1029/2008GL034536, 2008.
Yang, J., Xiao, C., Liu, J., Li, S., and Qin, D.: Variability of Antarctic sea ice extent over the past 200 years, Sci. Bull., 66, 2394–2404, 2021.
Yeung, N. K.-H., Menviel, L., Meissner, K. J., and Sikes, E. L.: Assessing he spatial origin of Meltwater Pulse 1A using oxygen-isotope fingerprinting, Paleoceanogr. Paleoclimatol., 34, 2031–2046, 2019.
Yuan, N., Ding, M., Ludescher, J., and Bunde, A.: Increase of the Antarctic Sea Ice Extent is highly significant only in the Ross Sea, Sci. Rep., 7, 41096, https://doi.org/10.1038/srep41096, 2017.
Yuan, X.: ENSO-related impacts on Antarctic sea ice: A synthesis of phenomenon and mechanisms, Ant. Sci., 16, 415–425, 2004.
Zielinski, U. and Gersonde, R.: Diatom distribution in Southern Ocean surface sediments (Atlantic sector): Implications for paleoenvironmental reconstructions, Palaeogeogr. Palaeoclimatol. Palaeoecol., 129, 213–250, 1997.
Zielinski, U., Gersonde, R., Sieger, R., and Fütterer, D.: Quaternary surface water temperature estimations: Calibration of a diatom transfer function for the Southern Ocean, Paleoceanography, 13, 365–383, 1998.
Zunz, V., Goosse, H., and Massonnet, F.: How does internal variability influence the ability of CMIP5 models to reproduce the recent trend in Southern Ocean sea ice extent?, The Cryosphere, 7, 451–468, https://doi.org/10.5194/tc-7-451-2013, 2013.
Zwally, H. J., Comiso, J. C., Parkinson, C. L., Cavalieri, D. J., and Gloersen, P.: Variability of Antarctic sea ice 1979–1998, J. Geophys. Res.-Oceans, 107, 3041, https://doi.org/10.1029/2000JC000733, 2002.
- Abstract
- Introduction
- Modern sea-ice formation and trends in the Southern Ocean
- Proxies for past sea-ice changes
- Past sea-ice changes
- Future directions
- Data availability
- Author contributions
- Competing interests
- Disclaimer
- Special issue statement
- Acknowledgements
- Financial support
- Review statement
- References
- Supplement
- Abstract
- Introduction
- Modern sea-ice formation and trends in the Southern Ocean
- Proxies for past sea-ice changes
- Past sea-ice changes
- Future directions
- Data availability
- Author contributions
- Competing interests
- Disclaimer
- Special issue statement
- Acknowledgements
- Financial support
- Review statement
- References
- Supplement