the Creative Commons Attribution 4.0 License.
the Creative Commons Attribution 4.0 License.
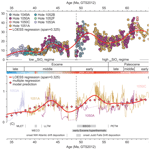
North Atlantic marine biogenic silica accumulation through the early to middle Paleogene: implications for ocean circulation and silicate weathering feedback
Jakub Witkowski
Karolina Bryłka
Steven M. Bohaty
Elżbieta Mydłowska
Donald E. Penman
Bridget S. Wade
The Paleogene history of biogenic opal accumulation in the North Atlantic provides insight into both the evolution of deepwater circulation in the Atlantic basin and weathering responses to major climate shifts. However, existing records are compromised by low temporal resolution and/or stratigraphic discontinuities. In order to address this problem, we present a multi-site, high-resolution record of biogenic silica (bioSiO2) accumulation from Blake Nose (ODP Leg 171B, western North Atlantic) spanning the early Paleocene to late Eocene time interval (∼65–34 Ma). This record represents the longest single-locality history of marine bioSiO2 burial compiled to date and offers a unique perspective into changes in bioSiO2 fluxes through the early to middle Paleogene extreme greenhouse interval and the subsequent period of long-term cooling. Blake Nose bioSiO2 fluxes display prominent fluctuations that we attribute to variations in sub-thermocline nutrient supply via cyclonic eddies associated with the Gulf Stream. Following elevated and pulsed bioSiO2 accumulation through the Paleocene to early Eocene greenhouse interval, a prolonged interval of markedly elevated bioSiO2 flux in the middle Eocene between ∼46 and 42 Ma is proposed to reflect nutrient enrichment at Blake Nose due to invigorated overturning circulation following an early onset of Northern Component Water export from the Norwegian–Greenland Sea at ∼49 Ma. Reduced bioSiO2 flux in the North Atlantic, in combination with increased bioSiO2 flux documented in existing records from the equatorial Pacific between ∼42 and 38 Ma, is interpreted to indicate diminished nutrient supply and reduced biosiliceous productivity at Blake Nose in response to weakening of the overturning circulation. Subsequently, in the late Eocene, a deepwater circulation regime favoring limited bioSiO2 burial in the Atlantic and enhanced bioSiO2 burial in the Pacific was established after ∼38 Ma, likely in conjunction with re-invigoration of deepwater export from the North Atlantic. We also observe that Blake Nose bioSiO2 fluxes through the middle Eocene cooling interval (∼48 to 34 Ma) are similar to or higher than background fluxes throughout the late Paleocene–early Eocene interval (∼65 to 48 Ma) of intense greenhouse warmth. This observation is consistent with a temporally variable rather than constant silicate weathering feedback strength model for the Paleogene, which would instead predict that marine bioSiO2 burial should peak during periods of extreme warming.
- Article
(3822 KB) - Full-text XML
-
Supplement
(1776 KB) - BibTeX
- EndNote
Biogenic silica (bioSiO2) secretion by marine plankton and the subsequent accumulation of biosiliceous marine sediments represent the main output flux in the global silicon cycle (Tréguer and De La Rocha, 2013). The present-day silicon cycle is also closely linked to the carbon cycle because diatoms – the most successful and efficient bioSiO2-secreting plankton group in the modern oceans – are also the key marine primary producers, responsible for up to 40 % of total global net photosynthesis per year (Smetacek, 1999). Owing to the ballast effect of their siliceous valves, diatoms are extremely efficient at exporting organic carbon (Corg) from the surface to the deep ocean and facilitating Corg burial in marine sediments (Yool and Tyrrell, 2003). This relationship between the silicon and carbon cycles has profound implications for understanding climate change on both long and short timescales in the past, founded on the premise that sedimentary bioSiO2 mass accumulation rates (fluxes) represent the rate of bioSiO2 burial and, importantly, that the burial rate reflects bioSiO2 production in surface waters at the time of deposition (Ragueneau et al., 2000; Yool and Tyrrell, 2005).
One fundamental control on marine siliceous plankton production is the amount of dissolved silicon supplied to the oceans from terrestrial silicate weathering, a chemical process that ultimately consumes atmospheric CO2 and releases silicic acid and alkalinity to the oceans (i.e., when combined with carbonate burial in the oceans; Walker et al., 1981; Fontorbe et al., 2020; Penman et al., 2020). By moderating atmospheric CO2, the silicate weathering feedback is postulated to operate as a thermostat, maintaining the Earth's surface within a habitable range of temperatures since early in geological history (Kasting, 2019). In today's rapidly warming world, an accurate understanding of the operation of silicate weathering as a climate feedback mechanism is essential.
Past transient greenhouse warming events, such as the “hyperthermal” events of the early to middle Paleogene (Paleocene and Eocene epochs; ∼66–34 Ma), offer ancient points of comparison for the present-day warming and future climate scenarios. Existing studies suggest that Paleogene bioSiO2 accumulation patterns are directly linked to variations in continental weathering on both long (Muttoni and Kent, 2007; Cermeño et al., 2015; Renaudie, 2016) and short (Witkowski et al., 2014; Penman, 2016; Penman et al., 2019) timescales. Additionally, large volumes of diatomite and diatom-rich clays deposited on continental shelves during the early Paleogene (e.g., Oreshkina and Aleksandrova, 2007) suggest that the supply of dissolved silicon from continental weathering under greenhouse climates exerted a strong influence on marine bioSiO2 accumulation. These interpretations are based on an assumption that diatoms were already key players in global silicon and carbon cycling in the early Cenozoic (see Fontorbe et al., 2016; Conley et al., 2017). Testing this assumption using the diatom fossil record is problematic due to the vulnerability of diatom bioSiO2 to diagenetic alteration (see Witkowski et al., 2020b, for a discussion and references). Thus, most interpretations concerning the long-term silicon availability impact on marine diatom production, as well as most scenarios for the timing of diatom rise to ecological prominence, are based on a range of indirect evidence, including modeling, isotope (bio)geochemistry, statistical treatment of large databases, and insights from other biosilicifying groups (for a recent synthesis, see Hendry et al., 2018).
In the modern oceans, deepwater circulation also exerts a major control on marine bioSiO2 accumulation patterns throughout the ocean basins. Firstly, ocean circulation determines the distribution and concentration of limiting macronutrients (N, P, dissolved Si) in deep waters and their upwelling into surface waters where they fuel primary production, and, secondly, circulation impacts bioSiO2 preservation in seafloor sediments (Ragueneau et al., 2000). Most of the present-day biosiliceous production is focused along continental margins in areas where diatoms can take advantage of nutrients supplied from continental runoff and coastal upwelling (Malviya et al., 2016). A large proportion of bioSiO2, however, is recycled even before settling out of the photic zone (Van Cappellen et al., 2002), since the modern oceans are undersaturated with respect to SiO2 at all depths. Only a fraction of bioSiO2 produced in the photic zone therefore reaches the ocean floor, and, furthermore, only a fraction of the exported bioSiO2 is incorporated into sediments and preserved (Frings, 2017).
Both the strength of the silicate weathering feedback and ocean circulation patterns are believed to have undergone profound changes through the early to middle Paleogene as the Earth transitioned from a hothouse, ice-free climate state (e.g., Zachos et al., 2008; Kirtland-Turner et al., 2014; Anagnostou et al., 2016) to an icehouse climate marked by continental-scale ice sheets (Zachos et al., 2001; Miller et al., 2020). Isotopic weathering proxies ( and δ7Li) display a broad minimum spanning the late Paleocene through early Eocene interval (Misra and Froelich, 2012), which have been interpreted to indicate either flat continental relief through this period (and thus reduced rates of continental runoff; Froelich and Misra, 2015) or evidence for a variable strength of the negative feedback between climate and silicate weathering (Caves et al., 2016). Sea level fall associated with the onset of the Antarctic glaciation at the Eocene–Oligocene transition (EOT) (Zachos et al., 1996) and the intensification of the Himalayan orogeny are also thought to have altered the dominant weathering regime by facilitating physical rather than chemical weathering and by exposing large volumes of fresh rock to erosion and weathering (Cermeño et al., 2015).
The early Cenozoic was a time of low thermal gradients between surface and deep waters and between high and low latitudes, which limited vigorous overturning circulation (Moore et al., 2008; Vahlenkamp et al., 2018). There is, however, little consensus on the timing of the onset of production of Northern Component Water (NCW) sourced from the high-latitude North Atlantic, a precursor to today's North Atlantic Deep Water. Estimates for NCW onset vary from the late early Eocene (∼49–50 Ma; Hohbein et al., 2012) through the late Eocene (∼38 Ma; e.g., Borrelli et al., 2014; Coxall et al., 2018) to across the EOT interval (∼34 Ma; Via and Thomas, 2006; Abelson and Erez, 2017). Regardless, the long-term global cooling spanning the middle and late Eocene (∼48–34 Ma) is thought to have resulted in enhanced upwelling, and the opening of the Drake Passage is viewed as a milestone in establishing the global pattern of thermohaline circulation in its present-day form in the Atlantic (Via and Thomas, 2006; Katz et al., 2011; Borrelli et al., 2014; Abelson and Erez, 2017).
Despite the importance of siliceous biota in the present-day carbon cycle, our understanding of the temporal trends in marine bioSiO2 accumulation through the early Paleogene is limited. First-order observations indicate an association between peak chert–porcelanite occurrence and deepwater temperatures through the Early Eocene Climatic Optimum (EECO) (Muttoni and Kent, 2007; Witkowski et al., 2020b) and during short-lived hyperthermal events of the early Eocene (Penman et al., 2019). The rapid cooling at the end of the Eocene is also widely regarded as the period of diatom proliferation and diversification, especially in the Southern Ocean (Egan et al., 2013; Lazarus et al., 2014; Renaudie, 2016). However, trends in marine bioSiO2 accumulation in the period between these temporally broadly isolated events representing contrasting climate states are not well documented. The longest currently available perspective on marine bioSiO2 accumulation (Cretaceous through Miocene) is based on Deep Sea Drilling Project (DSDP) Leg 1 through 44 smear-slide data (i.e., data gathered between 1968 and 1978) converted to mass accumulation rates and binned into 10 Myr increments (Miskell et al., 1985). Direct sediment measurements of bioSiO2 concentrations (with calculated fluxes) through the Paleogene are sparse (e.g., Diester-Haass, 1995; Salamy and Zachos, 1999; Diekmann et al., 2004; Lyle et al., 2005; Iwasaki et al., 2014) and mostly focus on restricted time windows of the late Eocene through early Oligocene interval. A major reason for this is that bioSiO2 is highly vulnerable to water-column and seafloor dissolution, which results in early Paleogene siliceous phytoplankton occurrences often being confined to narrow stratigraphic intervals at many sites (see Barron et al., 2015; Witkowski et al., 2020b).
The observations summarized above provoke three fundamental questions. (1) How did bioSiO2 burial flux evolve through the early to middle Paleogene? (2) What were the main controls on changes in marine bioSiO2 burial in this time interval? (3) What was the bioSiO2 burial response to long-term Paleogene climate changes? Through the early Paleogene, bioSiO2 accumulation was largely focused in the Atlantic and on the Eurasian Platform (Miskell et al., 1985; Muttoni and Kent, 2007; Moore et al., 2008; Barron et al., 2015; Wade et al., 2020). Unusually expanded lower Paleocene through upper Eocene biosiliceous successions were recovered from Blake Nose in the midlatitude western North Atlantic (Shipboard Scientific Party, 1998a–f; Witkowski et al., 2020a). In order to gain a quantitative insight into how bioSiO2 burial evolved through the early Paleogene hothouse and the ensuing period of global cooling, we have generated a composite high-resolution Blake Nose bioSiO2 flux record from ∼65 to 34 Ma, spanning nearly the entire Paleocene and Eocene epochs. This work follows on from two previous publications with a focus on the Blake Nose early to middle Paleogene siliceous microfossils: (1) Witkowski et al. (2020a), in which a revised chronological framework is proposed for Sites 1050 and 1051; and (2) Witkowski et al. (2020b), in which Paleogene trends in chert and porcelanite occurrences are compared to spatial and temporal patterns in Atlantic biosiliceous sediment occurrences. In our study here, we aim to determine the main controls on bioSiO2 fluxes in a key locus of biosiliceous accumulation in the western North Atlantic (Blake Nose) during the early to middle Paleogene – a period of Cenozoic climate change characterized by profound variations in global temperature.
2.1 Study sites and stratigraphy
This study is focused on drill cores recovered as part of the Ocean Drilling Program (ODP) Blake Nose Paleoceanographic Transect and includes Holes 1049A, 1050A/C, 1051A, 1052B/F, and 1053A (Shipboard Scientific Party, 1998b–f) (Fig. 1) (Table 1). The transect was drilled on Blake Nose (BN; also often referred to as “Blake Ridge”) in the western North Atlantic Ocean in order to reconstruct the Cretaceous–Paleogene paleoceanographic history of the region adjacent to the South Atlantic Bight (Shipboard Scientific Party, 1998a), offshore of the southeastern US seaboard between Florida Straits and Cape Hatteras (Gula et al., 2016). The BN is a northeast-trending extension of the Blake Plateau comprised of a Cretaceous to Paleogene continental margin succession (Pinet et al., 1981; Shipboard Scientific Party, 1998a) (Fig. 1b). As Paleogene sediments draping BN were deposited on the seaward slope of a large reef formed in the Early Cretaceous, the transect sites likely retain the relative depths of the paleo-reef system (Shipboard Scientific Party, 1998a). Drilling at BN, as well as at sites further north along the North American margin, documented well-preserved early Paleogene siliceous microfossils (e.g., Gombos, 1982; Nishimura, 1992; Hollis, 2014). The BN transect sites that recovered the most expanded early to middle Paleogene sections (Sites 1050 and 1051) include only a few narrow chert and porcelanite-bearing intervals (Witkowski et al., 2020b) and sparse clinoptilolite (a zeolite alteration product of biogenic silica) occurrences, which suggests minimal diagenesis of sedimentary bioSiO2. As such, the good overall preservation of siliceous microplankton in Paleogene sediments at BN, combined with the exceptionally long stratigraphic span of the record recovered during Leg 171B (Witkowski et al., 2020a, and references therein), makes the BN transect especially well-suited for reconstructing variations in bioSiO2 burial flux in the early Paleocene through late Eocene time period. To this end, we examined 1230 samples from five BN drill sites: Sites 1049, 1050, 1051, 1052, and 1053.
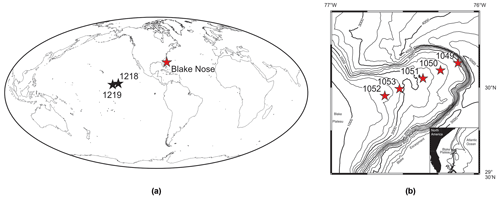
Figure 1Maps showing the location of sites considered in the present paper: (a) Blake Nose (red star) in the western North Atlantic and eastern equatorial Pacific sites (black stars). Base map generated using Ocean Drilling Stratigraphic Network (2021) Advanced Plate Tectonic Reconstruction service (https://www.odsn.de/, last access: 12 April 2021). (b) Location of the Ocean Drilling Program (ODP) Leg 171B sites on Blake Nose. Modified from Shipboard Scientific Party (1998a).
Table 1Sites included in this study, along with geographic coordinates, site chapters, and number of samples examined for bioSiO2. Sites used for bioSiO2 flux calculations are in italics.
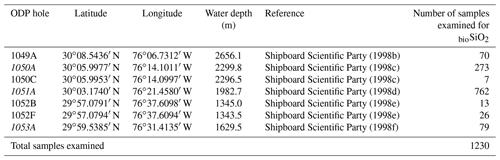
Site 1049 is the most distal and deepest site included in this study (1000–2000 m paleodepth; Shipboard Scientific Party, 1998b) (Fig. 1b; Table 1). The Paleogene section of Hole 1049A was poorly recovered due to the extensive presence of chert horizons. As a consequence, numerous biostratigraphic datums are poorly constrained through the recovered sequence, and age control is only approximate, especially through the early–middle Eocene transition (EMET). Based on the recent revisions to the bio-magnetostratigraphy of Holes 1050A and 1051A, however, Witkowski et al. (2020b) proposed a revised age model for the Paleocene through Eocene interval of Hole 1049A. Here, we examine 70 samples from the chert-free, siliceous-microfossil-bearing interval of Hole 1049A, spanning Cores 1049A-3H through -12X (∼21.1 to 88.1 compacted meters below seafloor; m b.s.f. – see Witkowski et al., 2020a; Table 1). Due to incomplete recovery and the temporal patchiness of the record, however, we do not include data from Hole 1049A in bioSiO2 flux calculations.
Site 1050 (1000–2000 m paleodepth; Shipboard Scientific Party, 1998c) was drilled several kilometers upslope of Site 1049 (Fig. 1b; Table 1). The Paleogene succession cored in Holes 1050A and 1050C is considerably more expanded and stratigraphically more complete than in Hole 1049A. Siliceous microfossils occur throughout the succession cored in Hole 1050A, but in Hole 1050C siliceous microfossils are confined to Core 1050C-2R (Witkowski et al., 2020b). We apply the age model of Witkowski et al. (2020a), who interpreted the presence of two major stratigraphic gaps. For this study, we examined 273 samples from Hole 1050A (Cores 1050A-2H through -36X; ∼11 to 319.3 compacted m b.s.f.) and 7 samples from Hole 1050C (Core 1050C-2R; 328 to 336 compacted m b.s.f.).
Site 1051, the intermediate-depth site of the BN transect (1000–2000 m paleodepth; Shipboard Scientific Party, 1998d) (Fig. 1b; Table 1), recovered the most expanded lower Paleocene through upper Eocene succession among Leg 171B sites. Siliceous microfossils occur throughout this succession, except for within several narrow dissolution intervals (for details see Witkowski et al., 2020b). We use the age model of Witkowski et al. (2020a), who showed that the Hole 1051A succession is interrupted by two major gaps that are broadly correlative with the hiatuses in Hole 1050A (see also Röhl et al., 2003). A total of 762 samples from the entire succession cored at Hole 1051A (∼8.5 to 644 compacted m b.s.f.) were examined for this study.
Site 1052 is the shallowest site of the BN transect sites, drilled near the crest of the BN (Fig. 1b; Table 1). Most of the middle bathyal (600–1000 m paleodepth; Shipboard Scientific Party, 1998e) Paleogene succession at this site is truncated by a prominent hiatus. In this study, we include a narrow composite interval of Holes 1052B and 1052F (∼77 to 131 meters composite depth, mcd) spanning the Middle–Late Eocene Turnover (MLET) (Kamikuri and Wade, 2012). As this interval overlaps parts of Holes 1051A and 1053A, it is not considered in sediment flux calculations. For age control at Site 1052, we use the bio-magnetostratigraphic constraints from Shipboard Scientific Party (1998e), Ogg and Bardot (2001), and Wade et al. (2012), following Witkowski et al. (2020b). A total of 39 samples from Site 1052 were used in this study.
Site 1053 was drilled between Sites 1051 (intermediate depth) and 1052 (shallowest depth) in the upper part of the BN transect (500–700 m paleodepth; Shipboard Scientific Party, 1998f) (Fig. 1b; Table 1). Site 1053 recovered an expanded siliceous-microfossil-rich upper Eocene section with no detectable stratigraphic gaps, as indicated by the age model of Borrelli et al. (2014). At total of 79 samples from Hole 1053A (∼0.5 to 183 meters below seafloor, m b.s.f.) were examined for this study.
Despite two major discontinuities and multiple recovery gaps, our composite BN record is comprised of data from five sites and spans the earliest Paleocene (∼64.74 Ma; magnetochron C28n in Hole 1051A, Witkowski et al., 2020a) through latest Eocene (∼33.94 Ma; magnetochron C13r in Hole 1053A; Borrelli et al., 2014) interval. This composite represents the longest currently available single-locality record of deep-sea biosiliceous sedimentation through the Paleogene. We report all ages relative to the Gradstein et al. (2012) timescale, hereafter referred to as GTS2012.
2.2 bioSiO2 measurements
bioSiO2 concentrations were determined by means of a Hach DR-3900 spectrophotometer using Hach method 8186 (heteropoly blue method). All spectrophotometric analyses closely followed the wet alkaline extraction procedure of Olivarez Lyle and Lyle (2002). Unlike Olivarez Lyle and Lyle (2002), however, for base extraction we used 1 M KOH and 10 mg ground sediment subsamples rather than 2 M KOH and 20 mg subsamples. This was done in order to avoid SiO2 polymerization (Annette Olivarez Lyle, personal communication, 2015), manifested by the precipitation of whitish filaments in test tubes following base extraction conducted at higher KOH concentrations with larger subsamples. bioSiO2 concentrations for individual sites are tabulated in Tables S1 through S5 in the Supplement, and data used for bioSiO2 flux calculations are presented in Figs. S1–S3 in the Supplement.
Three methods were employed to monitor analytical precision of the bioSiO2 measurements: (1) one sample in each analyzed batch was subject to stepwise standard addition against a target curve using liquid SiO2 standard supplied by Hach (average target curve R2=0.994, n=84); (2) one random sample from every sample batch was also analyzed in duplicate, with good correlation between duplicate analyses (average R2=0.98, n=92; Fig. S4); and (3) one of three in-house consistency standards was analyzed in approximately every second sample batch.
2.3 Sediment mass accumulation rate calculations
All sediment mass accumulation rate (hereafter: flux) values in this work are expressed as grams per square centimeter (g cm−2) per 1000 years (kyr) and are calculated using standard terms from previous studies (e.g., Diester-Haass, 1995; Piela et al., 2012; D'haenens et al., 2014).
Use of magneto-biostratigraphic age models to establish LSRs typically produces unrealistic jumps in sediment flux estimates at magnetostratigraphic boundaries, with order-of-magnitude differences between consecutive age–model tie points. In order to smooth out such abrupt features, which we deem to be artifacts of the applied age models, in our flux records, we fitted polynomial regressions against the age vs. depth curves (or segments thereof comprised between hiatuses), following the approach of Piela et al. (2012). The datasets developed in the present work are based on several holes that include several hiatuses, which is why robust age models that are consistent between holes are essential to obtain a reliable composite stratigraphy. We therefore plot the flux records derived from smoothed LSR estimates using ages interpolated from the original (i.e., non-smoothed) age–depth curves (Figs. S1–S3).
Sediment flux studies often estimate wet bulk sediment density through calibration of high-resolution estimates of wet bulk density (obtained via gamma ray attenuation, GRA, analysis) against discrete DBD measurements collected during routine shipboard analysis. In the present work, establishing a single GRA–DBD correlation over the entire cored interval proved ineffective for Sites 1050 and 1051, likely due to the downhole increase in compaction. Instead, we estimated DBD for a given depth by interpolating between shipboard discrete DBD measurements (Shipboard Scientific Party, 1998b–d, f). Sediment density plots, LSRs, and calculated fluxes are included in the Supplement (Figs. S1–S3 and Tables S2–S3, S5).
2.4 Stable isotope and pCO2 data
Our interpretation of possible controls on early to middle Paleogene bioSiO2 accumulation is based on comparison to published isotopic weathering (, , and δ7Li; Ravizza et al., 2001; Ravizza and Peucker-Ehrenbrink, 2003; Misra and Froelich, 2012; Klemm et al., 2005) and paleocirculation proxies (δ13C, δ18O; Cramer et al., 2009), as well as a recent atmospheric pCO2 reconstruction (Foster et al., 2017) and silicate weathering flux model (hereafter SWF) (Caves et al., 2016). For further discussion and a full documentation of data sources see the Supplement (“Stable isotope and pCO2 data” section and Fig. S5).
2.5 Statistical treatment
Smoothed long-term trends in bioSiO2 flux and published geochemical records were obtained via local regression (abbreviated as LOESS; Cleveland et al., 1992) computed using R Studio v. 3.5.1. Statistical analysis was performed on smoothed time series (bioSiO2 flux, δ13C, δ18O, pCO2 , , δ7Li, and SWF) using Statistica 13.1 package.
The degree of covariance of the analyzed variables was assessed by correlation analysis. A normality test procedure was carried out for all variables using the Shapiro–Wilk test (α=0.05). The Pearson correlation coefficient was used to assess covariance for each pair of variables characterized by a normal distribution. The nonparametric Spearman correlation coefficient was used when non-normal distribution was indicated for a given variable by the Shapiro–Wilk test.
The analysis also involved a multiple regression model, which describes the relationship of the dependent variable Y with a set of independent variables X1, (which, in this study, is the relationship between bioSiO2 flux and other proxy records). It is defined by Eq. (1):
where βj represents model parameters (regression coefficient), and ξ is a random component. The parameters of the regression equation are estimated using the least-squares method, and the determination coefficient and standard error of estimation are used to assess the goodness of the model.
Our new composite %bioSiO2 record from Blake Nose spans the interval between ∼65 and 34 Ma (Fig. 2a), representing the longest single-locality record of bioSiO2 concentrations compiled to date. The composite record, however, lacks data in two short time windows: between ∼53.5 and 52.0 Ma (magnetochrons C24n through C23n) and between ∼47.5 and 49.0 Ma (i.e., through the EMET). This is due to the presence of prominent hiatuses at all study sites spanning these intervals (Shipboard Scientific Party, 1998b–d; Witkowski et al., 2020a) (Fig. 2a).
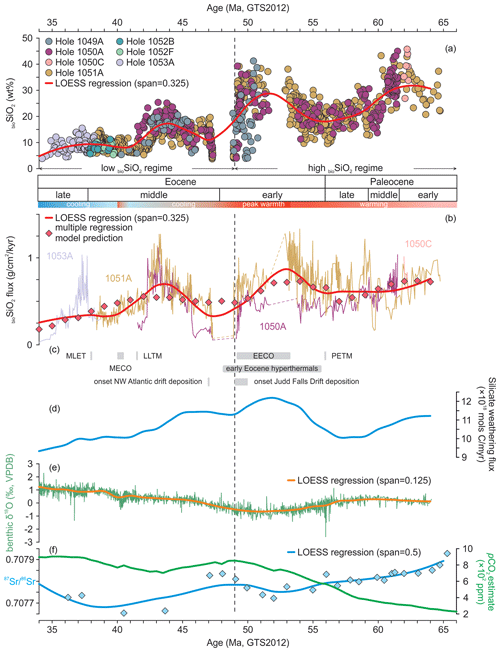
Figure 2Composite Blake Nose weight percent biogenic silica concentrations (a) and fluxes (b) through the early to middle Paleogene plotted against key tectonic and climatic events (c), silicate weathering flux as modeled by Caves et al. (2016) (d), global benthic foraminiferal δ18O compilation of Cramer et al. (2009; rescaled to GTS2012) (e), and pCO2 reconstruction (Foster et al., 2017) and ratios (Misra and Froelich, 2012) (f). The schematic representation of climatic trends next to the chronostratigraphy panel is consistent with Cramwinckel et al. (2018). Abbreviations: wt % – weight percent; bioSiO2 – biogenic silica; GTS2012 – Geologic Time Scale 2012; see Gradstein et al. (2012).
The BN %bioSiO2 composite shows variable but generally high values between ∼65 and 49 Ma (Fig. 2a). Two broad %bioSiO2 maxima are observed within this high-bioSiO2 interval, culminating at ∼61.5 Ma and at ∼51.5 Ma (Fig. 2a). These maxima are separated by a broad low in %bioSiO2 with a nadir centered at approximately the Paleocene–Eocene boundary (∼56 Ma). From ∼49 Ma to the end of the record at ∼34 Ma, %bioSiO2 levels are considerably lower and less variable (Fig. 2a), with a distinct maximum culminating at ∼44 Ma.
Long-term trends in BN bioSiO2 fluxes are calculated based on a composite record built from datasets generated from Sites 1050, 1051, and 1053. Through the Paleocene and early Eocene, Site 1050 generally displays lower bioSiO2 fluxes than Site 1051 (Fig. 2b). From ∼46 to 34 Ma, both bioSiO2 flux trends and values are remarkably consistent between Sites 1050 and 1051 (Fig. 2b). The short time interval in which records from Site 1051 and Site 1053 overlap also reveals coherent bioSiO2 flux values (Fig. 2b). Thus, following a period of high inter-site variability through the Paleocene, three intervals of elevated bioSiO2 fluxes are observed, which are consistent between sites and peak at ∼53.2, ∼43.3, and ∼37.7 Ma. The overall patterns in %bioSiO2 and bioSiO2 flux estimates are also consistent, especially through the middle and late Eocene. Most importantly, however, the bioSiO2 flux values fall within the same order of magnitude through most of the study period (except for peak bioSiO2 fluxes at Site 1051 between 54 and 53 Ma and from 44 to 43 Ma). Furthermore, our record consistently shows that bioSiO2 fluxes through the middle Eocene cooling were, on average, higher than (Site 1050) or similar to (Site 1051) bioSiO2 fluxes through the early Eocene period of extreme greenhouse warmth (Fig. 2b).
3.1 Impact of hiatuses and diagenesis on BN bioSiO2 flux estimates
The bioSiO2 records from Sites 1050 and 1051, which constitute the older part of the composite presented here, are interrupted by hiatuses. These discontinuities in the BN record could introduce a bias to the flux estimates, for instance by influencing the LSR calculations. The age models for Holes 1050A/C and 1051A used in this study (see Witkowski et al., 2020a, for details), however, are highly consistent in that the hiatuses are identified in correlative intervals, and, furthermore, LSRs used in flux calculations were subjected to polynomial smoothing, which should eliminate most short-term artifacts imposed by age model imperfections. bioSiO2 flux estimates could also be compromised by winnowing, which could concentrate biosiliceous particles over some areas of the seabed, while removing them from adjacent areas. In the core description logs for BN sites included in the present bioSiO2 flux reconstruction, explicit mention of winnowing is made only in one instance, i.e., for Core 1051A-41X (Shipboard Scientific Party, 1998d). This core is also characterized by the abundant presence of zeolite crystals (likely clinoptilolite; Jakub Witkowski, unpublished observations), which are an indicator of bioSiO2 diagenesis (see Fenner, 1991). For this reason, Core 1051A-41X was excluded from the present study. Also, %bioSiO2 measurements were not performed on the sparse cherty or porcellanic intervals at Sites 1050 and 1051. Scanning electron microscope examination of diatoms from the remaining intervals of the BN composite indicates only minor diagenetic effects on the siliceous microfossils, manifested mostly by dissolution of the most delicate parts of the valves, such as areole occlusions or pore fields. For these reasons, the bioSiO2 fluxes reconstructed in this study are deemed to be robust.
3.2 Controls on bioSiO2 accumulation through the early to middle Paleogene at Blake Nose
bioSiO2 production, export, and preservation in marine sediments are influenced globally by dissolved silicon supply to the oceans derived from terrestrial weathering, which is closely linked to climate via a negative feedback (e.g., Walker et al., 1981), and by ocean circulation patterns and upwelling, which supply the bulk of macronutrients to surface waters (Miskell et al., 1985; Handoh et al., 2003). In order to gain insight into the influence that each of these factors has exerted on bioSiO2 accumulation through the early to middle Paleogene at BN, we compared the bioSiO2 flux composite record to published composite global benthic foraminiferal δ18O and δ13C records, pCO2 proxy estimates, proxy records of continental weathering (, and δ7Li), and modeled silicate weathering flux (SWF) (Figs. 2, S5).
We find that bioSiO2 flux is moderately correlated with modeled SWF (r=0.597, p<0.05) and more strongly, but inversely, correlated with both pCO2 (, p<0.05) and δ18O (, p<0.05) (Fig. 3). A weaker, but still statistically significant, correlation exists between bioSiO2 flux and δ7Li (, <0.05), (r=0.430, p<0.05), and (0.418, p<0.05) (Fig. 3). No statistically significant correlation has been found between bioSiO2 flux and trends in benthic foraminiferal δ13C. These relationships suggest that, overall, through the early to middle Paleogene, bioSiO2 flux at BN was indirectly shaped by a combination of changes in atmospheric greenhouse gas levels, bottom water temperatures (assuming ice-free poles through our study period), and supply of solutes from terrestrial silicate weathering.
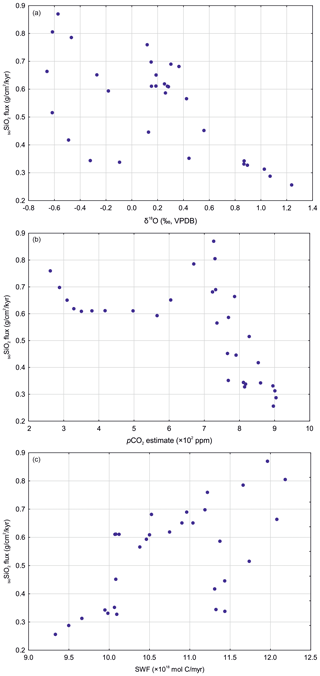
Figure 3Correlation scatter plots for the three strongest statistical relationships identified in the present study: biogenic silica flux vs. δ18O (a), pCO2 (b), and silicate weathering flux (c). Abbreviations: bioSiO2 – biogenic silica; SWF – silicate weathering flux. Data sources indicated in the text.
Multiple regression indicates four significant variables shaping BNbioSiO2 flux: δ18O, pCO2, δ13C, and . Except for δ13C, this is consistent with the correlations discussed above. Notably, SWF was excluded by the multiple regression model. This is likely due to the high overall similarity in temporal trends displayed by BN bioSiO2 flux and SWF. The multiple regression model equation takes the form
This model explains ∼71 % of BN bioSiO2 flux variance, with a standard error of estimation equal to 0.09. We find that the model reproduces our calculated bioSiO2 flux values reasonably well (Fig. 2b), suggesting that the use of smoothed datasets is suitable for identifying long-term trends in bioSiO2 fluxes. Thus, both the correlations and multiple regression suggest that BN bioSiO2 flux was shaped mostly by δ18O, pCO2, and the supply of continental weathering products – all of which are related to the temperature–silicate weathering feedback.
4.1 Implications for paleocirculation
The Blake Nose area is positioned on the western margin of the North Atlantic subtropical gyre, which exerts a major control on nutrient availability in surface waters along the North American continental margin (Pelegrí et al., 1996). Over the South Atlantic Bight region, encompassing the Blake Plateau, the key mechanism fueling modern phytoplankton production is sub-mesoscale frontal eddies arising from meanders on the landward side of the Gulf Stream System (GSS; Richardson, 2001; Gula et al., 2015). Comparable cyclonic eddies of <100 km diameter are also observed in other western boundary current (WBC) systems, which are generally viewed as oligotrophic settings (Roughan et al., 2017). These eddies are responsible for upward pumping of nutrients from sub-thermocline, nitrate-rich waters (Lee et al., 1991). Upwelled waters intrude onto the continental margin and sustain rich biological production through the lifespan of an eddy (Roughan et al., 2017). Siliceous plankton production and export in the GSS is influenced by a number of factors, including Atlantic Meridional Overturning Circulation (AMOC) intensity and the North Atlantic Oscillation, which together act to shift the GSS position relative to the North American seaboard on a decadal timescale (Sanchez-Franks and Zhang, 2015). Also, the topography of the North American continental margin (Richardson, 2001) in conjunction with eustatic sea level variations exert a strong influence on the GSS path on long timescales, with features such as the Charleston Bump acting to deflect the jet trajectory toward the open ocean (Pinet et al., 1981; Gula et al., 2015).
A northeastward-flowing, wind- and Coriolis-force-driven WBC likely operated in the North Atlantic at least since the Cretaceous (Gradstein and Sheridan, 1983), albeit at reduced strength relative to the modern era before the final closure of the Central American Seaway (Montes et al., 2012). Given the overall stability of the western North Atlantic topography over the Cenozoic, cyclonic frontal eddies were likely an inherent feature of the South Atlantic Bight region throughout the Paleocene and Eocene. The semi-periodic fluctuations in BN bioSiO2 flux through time could therefore also be attributed to changes either in the mean GSS path (e.g., Wade and Kroon, 2002) or variations in sub-thermocline nutrient supply, which are largely dependent on vertical mixing of the ocean (Miskell et al., 1985; Moore et al., 2008) – or a combination of both processes.
Reconstructing intermediate-water and deepwater circulation patterns in the North Atlantic through the early Cenozoic is more complex than reconstructing GSS history. Vahlenkamp et al. (2018) reviewed the existing perspectives on the Atlantic Ocean circulation through the Paleogene. εNd reconstructions generally indicate a southern high-latitude source for the deep waters bathing the North American margin throughout the early to middle Paleogene (Thomas et al., 2003; Batenburg et al., 2018), although a Tethyan-sourced water mass is also hypothesized by some researchers (Fontorbe et al., 2016; Vahlenkamp et al., 2018). At present, it is not known how a southern-sourced, northward-flowing deepwater mass may have affected nutrient availability and upwelling in the western North Atlantic, especially along continental margins. The high diatom : radiolarian (D:R) ratios (Witkowski et al., 2020b, for further discussion see below) and common occurrence of well-preserved epiphytic diatoms such as Arachnoidiscus (see Witkowski et al., 2020a) suggest that much of the BN bioSiO2 flux through the Paleocene may be attributed to neritic production. Varying proportions of continental-runoff-derived vs. upwelled nutrient input could also be invoked to explain the disparity in bioSiO2 fluxes between the more proximal Site 1051 and the more distal Site 1050 through the Paleocene.
An intensely debated question in the early Paleogene deepwater circulation reconstructions is the timing of the onset of NCW flow – a precursor to quasi-modern deepwater circulation (Via and Thomas, 2006). North Atlantic δ13C records do not indicate major paleocirculation changes prior to the late Eocene (∼38 Ma; Katz et al., 2011; Borrelli et al., 2014; Coxall et al., 2018), and numerous studies place the onset of AMOC either shortly prior to or following the EOT (Via and Thomas, 2006; Abelson and Erez, 2017; Coxall et al., 2018).
In contrast to the timing of NCW flow initiation indicated by isotopic proxy records, the onset of widespread drift deposition in the North Atlantic is documented considerably earlier, i.e., near the termination of the EECO (∼49 Ma; Hohbein et al., 2012; Boyle et al., 2017). This is also synchronous with ubiquitous deep-sea erosion coincident with the EMET (Aubry, 1995; Witkowski et al., 2020b), strongly suggesting that the onset of vigorous northern-sourced bottom current activity began at ∼49–47 Ma (Vahlenkamp et al., 2018; Witkowski et al., 2020a). Following the EMET, the northward-flowing GSS and the invigorated deep WBC facilitated diapycnal mixing, which likely enhanced biological pump efficiency along continental margins of the western North Atlantic. This is consistent with a range of geochemical proxies, including thallium isotope (ε205Tl) evidence for increased Corg burial from ∼50 Ma (Nielsen et al., 2009) and with surface-to-deep δ13C gradients (Hilting et al., 2008). BN diatom assemblage data from Witkowski et al. (2020b) also support an oligotrophic regime over BN for the time period prior to and including the EECO based on high percentages of hemiauloids. Following the EECO (after ∼49 Ma), elevated percentages of diatom resting spores point to alternating, perhaps seasonal, periods of nutrient enrichment and depletion, in line with strong periodic upwelling of nutrients by means of Gulf Stream frontal eddies (Lee et al., 1991). This interpreted invigoration in ocean mixing led to a considerable increase in primary production during the early middle Eocene, as evidenced by a rapid increase in both CaCO3 and bioSiO2 fluxes at BN at ∼46 Ma (Fig. 4a, b).
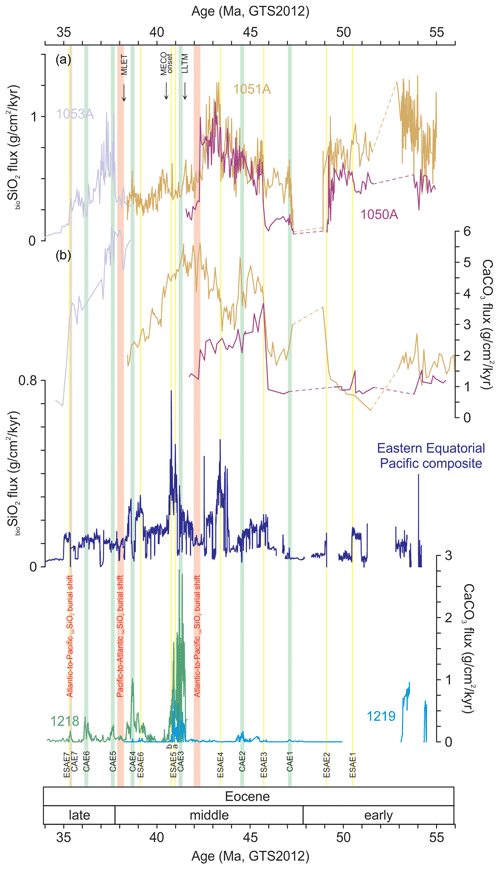
Figure 4Eocene biogenic silica (a) and calcium carbonate (b) fluxes at Blake Nose sites plotted against biogenic silica (c) and calcium carbonate (d) fluxes at eastern equatorial Pacific sites. Blake Nose carbonate data are from Shipboard Scientific Party (1998c, d, f). Eastern equatorial Pacific data are from Moore et al. (2008) and Lyle et al. (2005). Abbreviations: bioSiO2 – biogenic silica; GTS2012 – Geologic Time Scale 2012 (see Gradstein et al., 2012); MLET – Middle–Late Eocene Turnover; LLTM – Late Lutetian Thermal Maximum; ESAE – Eocene silica accumulation event; CAE – carbonate accumulation event.
4.2 Comparison to eastern equatorial Pacific bioSiO2 flux records
The only published early Paleogene bioSiO2 flux record from another region of comparable duration to the BN composite record is that derived from eastern equatorial Pacific (EEP) cores (Moore et al., 2008). There are several important differences between the Atlantic and Pacific records (Fig. 4), including (1) contrasting proportions of diatoms in the BN vs. EEP sediments, (2) the presence or absence of exported neritic material, and (3) a shorter time interval covered by the EEP record (Eocene only). Where the records overlap in time, however, there is a variable degree of coupling between bioSiO2 flux records from BN and EEP.
High diatom : radiolarian (D:R) ratios in early to middle Paleogene sediments at BN were interpreted by Witkowski et al. (2020b) to indicate that preserved bioSiO2 was mostly of diatom origin. It is challenging, however, to provide a quantitative estimate of the diatom vs. radiolarian contribution to total bioSiO2 at BN, primarily because no published diatom valve weight data are available. In the modern oceans, radiolarian tests are on average an order of magnitude heavier than diatom valves (with differences in fact ranging over several orders of magnitude; Lisitzin, 1971). Assuming a 10:1 radiolarian-to-diatom skeleton weight ratio for the early Paleogene and using an average radiolarian test weight of 0.225 µg consistent with the range of values displayed by the oldest materials included in Moore (1969), we make a rough estimate of the diatom vs. radiolarian contribution to total BN bioSiO2 based on quantitative siliceous microfossil counts of Witkowski et al. (2020b). As other siliceous plankton groups are sparse in BN siliceous microfossil assemblages (Witkowski et al., 2020b) and likely contribute little bioSiO2 to sediments (Lisitzin, 1971), we exclude the relatively minor contributions of silicoflagellates, siliceous dinoflagellates, and chrysophycean cysts from the calculations. These rough approximations indicate a mean diatom contribution of ∼35.7 % to the total biogenic silica content at BN (Fig. S6), with the highest values observed for the early and middle Paleocene, consistent with the D:R ratios ranging as high as >200 reported by Witkowski et al. (2020b). Given that diatom valves are less resistant to dissolution than radiolarian tests, the contribution of diatoms to total bioSiO2 in early Paleogene sediments at BN is likely underestimated due to selective dissolution.
It is important to note that these considerations disregard the contribution of siliceous sponge spicules to total bioSiO2 in the BN sediments. Counting and identifying sponge spicules were beyond the scope of the present study, and, to the best of our knowledge, no quantitative studies on sponge spicules from the BN cores have been performed thus far. Consequently, we were not able to use published data to estimate sponge spicule contribution to total bioSiO2 in early Paleogene sediments at BN. Several recent studies point to a declining contribution of sponges to the total bioSiO2 flux that is probably linked to diatom expansion in the late Mesozoic (Maldonado et al., 1999; Conley et al., 2017). Modern bioSiO2 flux attributed to sponges ranges from 25 to 48 Tg Si yr−1 and is an order of magnitude lower than the total bioSiO2 flux estimate for continental margins (140–235 Tg Si yr−1) and for the deep sea (153 Tg Si yr−1) (Hayes et al., 2020). Sponge spicules are therefore unlikely to have made a significant contribution to total bioSiO2 flux at BN through the early to middle Paleogene. SiO2 preservation is again a related issue, as sponge spicules undergo dissolution at slower rates compared to siliceous plankton valves or tests (Bertolino et al., 2017). Although some attempts have been made (e.g., Warnock and Scherer, 2015), there is currently no standardized quantitative measure of diatom preservation in sediments, and the basic indicators of silica dissolution are chert–porcelanite and clinoptilolite occurrences. As indicated above, both chert–porcelanite and clinoptilolite occur only at isolated, narrow levels at the sites included in bioSiO2 flux estimates in the present study, and in some intervals diatom preservation can be considered pristine. Hence, the assumption is that no extensive diatom silica dissolution has occurred in Holes 1050A and C and 1051A, which would lead to preferential preservation of the more dissolution-resistant sponge spicule silica over the more dissolution-prone diatom and/or radiolarian silica. Thus, our conclusion is that siliceous sponge spicules do not contribute significantly to total bioSiO2 flux in the BN cores.
In contrast to the abundant presence of diatoms at BN, Moore et al. (2008) refer to the near absence of diatoms in the Eocene EEP cores as an “enigma”. Although Moore et al. (2008) do not specify whether or not this observation is based on sieved residues (thereby potentially missing diatoms in the smaller sediment fractions), other Paleogene diatom reports from pelagic low-latitude Pacific sites corroborate this view (e.g., Fenner, 1984). We propose that the reason for this difference in diatom abundance in sediments between BN and EEP is twofold. Firstly, most early Paleogene diatom occurrences in the Atlantic are in marginal settings (Witkowski et al., 2020b), where at least part of the preserved diatom assemblage may originate from offshore export of neritic plankton, and diatom preservation may be fostered by higher concentrations of Al (DeMaster, 2014; Hayes et al., 2020). Secondly, the radiolarian-rich Pacific sites mostly represent pelagic deposition at water depths of ∼4–5 km. Diatom dissolution is facilitated by longer times in transit through the water column and longer times resting on the seafloor in slowly accumulating pelagic settings. The BN and EEP records also differ in the magnitude of bioSiO2 fluxes: as discussed in Witkowski et al. (2014, 2020b), the BN area received large volumes of neritic plankton through the early Paleogene, which underwent offshore export likely by means of frontal eddies, and the resultant bioSiO2 fluxes are high. The pelagic EEP sites likely record only local pelagic production and deposition, with low bioSiO2 fluxes relative to the BN.
Eocene EEP bioSiO2 accumulation rates are generally low, punctuated by a series of elevated flux events termed ESAEs (Eocene silica accumulation events – see Moore et al., 2008) and CAEs (carbonate accumulation events – see Lyle et al., 2005) (Fig. 4c–d). Between ∼55 and 46 Ma, bioSiO2 fluxes in the EEP are low and appear to be decoupled from the BN records. ESAE 3 at ∼45.8 Ma marks the onset of enhanced bioSiO2 flux in the EEP (Fig. 4c). Notably, ESAE 3 appears to be age-equivalent to a prominent increase in BN bioSiO2 and CaCO3 fluxes (Fig. 4a vs. c). ESAE 4 at ∼44.3 Ma is correlative with the peak in middle Eocene bioSiO2 flux at BN (Fig. 4a vs. c). Following ESAE 4, however, trends in bioSiO2 flux again become decoupled between the two regions. bioSiO2 fluxes diminish at BN between ∼42 and 38 Ma, and a concomitant reduction is observed in the geographic distribution of siliceous microfossils in the Atlantic Ocean (Witkowski et al., 2020b) (Fig. 4a vs. c). Thus, reduced bioSiO2 accumulation between ∼42 and 38 Ma is not a local phenomenon restricted to the BN area, but instead it is likely indicative of a major change in nutrient supply or paleocirculation that affected the entire Atlantic basin. In contrast, this late middle Eocene period of low bioSiO2 flux at BN precisely corresponds to an interval of elevated bioSiO2 fluxes in the EEP (Fig. 4a vs. c), including the bimodal ESAE 5, which represents the peak in the Eocene EEP bioSiO2 accumulation at ∼41 Ma. Thus, the decrease in nutrient levels in the Atlantic appears to have been associated with nutrient enrichment and elevated biosiliceous production in the EEP, representing an inter-basin shift in biosiliceous productivity and sedimentation.
Near the end of the middle Eocene, ESAE 6 is abruptly terminated at ∼38.5 Ma, concomitant with a major radiolarian turnover at EEP sites (Moore et al., 2008). A similar episode of accelerated turnover in radiolarians has been identified at ∼38.25 at BN (Kamikuri and Wade, 2012; Newsam et al., 2017), but in conjunction with a rapid rise in bioSiO2 flux levels. ESAEs 7 and 8 in the EEP are minor events at ∼35 and ∼34 Ma, respectively, and no age-equivalent events are observed in the BN bioSiO2 flux record. We interpret the decoupling between BN and EEP bioSiO2 flux records after ∼42 Ma as a series of inter-basin bioSiO2 accumulation shifts (Fig. 4a vs. c), likely associated with deepwater circulation changes affecting nutrient availability in surface waters, but likely also impacting the seabed preservation of bioSiO2 (Berger, 1970).
Diminished BN bioSiO2 fluxes indicate lower nutrient supply from ∼42 to 38 Ma, i.e., through the interval spanning both the Late Lutetian Thermal Maximum (LLTM, ∼41.5 Ma; Westerhold et al., 2018b) and Middle Eocene Climatic Optimum (MECO, ∼40 Ma; Bohaty et al., 2009; Henehan et al., 2020). Modern field observations indicate that diminished supply of nutrients to the GSS may result from weakened AMOC. Witkowski et al. (2020b) demonstrate a reduced geographic range of biosiliceous accumulation in the Atlantic between ∼42 and 38 Ma, which is also consistent with a diminished nutrient supply. Accordingly, we propose a period of potentially weakened AMOC spanning both the LLTM and MECO events. In the EEP, the high rates of primary production are sustained by advection of nutrient-rich sub-thermocline waters associated with the equatorial divergence (Fiedler et al., 1991). We propose that the paleocirculation changes that led to the interpreted disruption to AMOC may have manifested themselves by nutrient enrichment in the EEP. Thus, alternating loci of biosiliceous sedimentation between the Atlantic and Pacific during the middle Eocene likely resulted from circulation shifts that exerted control over bioSiO2 production and burial.
The Pacific-to-Atlantic bioSiO2 flux shift at ∼38 Ma coincides with increased rates of radiolarian turnover and planktonic foraminiferal extinction designated as MLET by Kamikuri and Wade (2012). Notably, the late Eocene is also believed to have been a period of pelagic diatom proliferation, probably due to the radiation of holoplanktonic taxa (Sims et al., 2006; Egan et al., 2013). Thus, MLET may have made a significant impact on siliceous microplankton evolution and production globally. Most importantly, however, the abrupt increase in bioSiO2 flux at Site 1053 shortly after MLET took place in conjunction with shifts in benthic foraminiferal δ13C (Katz et al., 2011; Borrelli et al., 2014; Coxall et al., 2018) interpreted to mark the onset of NCW export.
Thus, the repeated shifts in bioSiO2 fluxes between NW Atlantic and EEP through the late middle and late Eocene suggest that the “lagoonal” Atlantic (carbonate-burial-favoring) vs. “estuarine” Pacific (SiO2-burial-favoring) circulation pattern proposed by Berger (1970) was established in the lead-up to LLTM and temporarily reversed at the MLET before its final re-establishment shortly before the EOT. These shifts were likely driven by changes in deep-sea circulation patterns arising from both tectonic evolution in the Northern and Southern Hemisphere (e.g., Norwegian–Greenland Sea and Drake Passage region, respectively) and long-term Eocene climate change. Importantly, however, this interpretation implies that the two scenarios for NCW export inception (at EOT: Borrelli et al., 2014; Coxall et al., 2018; vs. at the end of the EECO: Hohbein et al., 2012; Boyle et al., 2017; Vahlenkamp et al., 2018) are not mutually exclusive. The patterns in Atlantic-to-Pacific bioSiO2 flux fractionation outlined above suggest a ∼4 Myr period of the early AMOC disruption spanning the last of the Eocene greenhouse warming events, i.e., LLTM and MECO. Moreover, the evidence supporting NCW inception in the late Eocene or early Oligocene may in fact point to a re-invigoration of AMOC flow following a period of weakened overturning circulation between 42 and 38 Ma.
4.3 Implications for the silicate weathering feedback operation mode
The silicate weathering feedback has been proposed as the key mechanism for keeping the Earth surface temperatures within a habitable range over 105–106-year timescales (Walker et al., 1981; Kasting, 2019). By consuming atmospheric CO2 and releasing alkalinity and dissolved silicon (Penman, 2016), this feedback mechanism also influences key biogeochemical cycles within the ocean–atmosphere system, resulting in a tight coupling between the marine carbon and silicon cycles (Tréguer and De La Rocha, 2013). In recent years, however, the operation of the silicate weathering feedback through the Cenozoic has been disputed, with a special focus on whether the strength of the link between climate and continental weathering varies through time (Caves et al., 2016; van der Ploeg et al., 2018). One point of disagreement concerns the early Paleogene. In the traditional view (hereafter “constant feedback strength scenario”), which assumes a linear relationship between global temperature change and weathering, the early Paleogene greenhouse climates should facilitate increased rates of chemical weathering on land directly proportional to the magnitude of CO2-driven warming (e.g., Misra and Froelich, 2012; Sluijs et al., 2013; Penman, 2016). An emerging alternative view (hereafter “variable feedback strength scenario”) is that during the Eocene the feedback strength was at a minimum level (Caves et al., 2016; van der Ploeg et al., 2018), with lowered silicate weathering intensity (and, hence, reduced weathering feedback strength) promoting high pCO2 levels and a warm climate (Misra and Froelich, 2012). Assuming that the early to middle Paleogene silicon cycle already operated in its present-day form (Fontorbe et al., 2016; Conley et al., 2017), these scenarios should lead to different marine bioSiO2 flux responses. In the constant feedback strength scenario, bioSiO2 production and burial would be expected to peak during the EECO, i.e., the warmest period of the Cenozoic era (e.g., Kirtland-Turner et al., 2014; Cramwinckel et al., 2018; Westerhold et al., 2018a). In the variable feedback strength scenario, the silicate weathering flux should decrease through the Eocene (Caves et al., 2016), leading to a decrease in dissolved silicon supply and bioSiO2 burial.
In the BN composite, both %bioSiO2 and bioSiO2 flux values are high in the lead-up to the EECO (∼55 through 53.5 Ma) and considerably lower in the final phases of the EECO (∼51 through 49 Ma). The hiatus spanning ∼53.5 through 52 Ma, however, precludes any definitive conclusions on the behavior of the silicate weathering feedback through the entire EECO period, particularly with regard to the constant vs. variable strength of its link to climate. However, bioSiO2 flux values through the middle Eocene are similar to or consistently higher than background Paleocene–early Eocene values in both the Atlantic and the Pacific (see also Moore et al., 2008, and Sect. 4.2), which cannot be easily reconciled with the linear feedback strength scenario. Thus, it appears that long-term trends in bioSiO2 flux are more consistent with the variable feedback strength scenario, suggesting that the strength of the link between climate and terrestrial silicate weathering may indeed be variable through time. Secondly, the high levels of bioSiO2 flux through the middle Eocene cooling (Fig. 2b) point to enhanced nutrient supply from invigorated ocean circulation as a major control on bioSiO2 flux in the younger part of our study period.
Deepwater temperatures, atmospheric greenhouse gas levels, and continental weathering are identified as the main drivers of bioSiO2 flux through the Paleocene and Eocene at Blake Nose in the western North Atlantic Ocean. Variations in bioSiO2 fluxes support an early export of NCW, but also suggest a period of disruption due to diminished AMOC between ∼42 and 38 Ma, as suggested by the Atlantic-to-Pacific bioSiO2 flux fractionation. NCW export likely became re-invigorated in the late Eocene, as indicated by a pulse of bioSiO2 flux and published paleocirculation proxy records. Additionally, BN bioSiO2 fluxes indicate that the long-term behavior of the silicate weathering thermostat conforms to the variable rather than constant weathering–climate feedback strength scenario.
Overall, this study also demonstrates that disentangling silicate weathering, productivity, and paleocirculation controls on bioSiO2 flux records is challenging. While the globally integrated flux of bioSiO2 to sediments must respond to global weathering rates, bioSiO2 flux records at individual sites do not necessarily reflect changes in the global flux because of site-specific or regional effects like circulation change. Our hope is that by continuing to develop bioSiO2 flux records from different parts of the oceans a comprehensive picture may emerge from future studies. These records will necessarily need to be constructed in conjunction with other lines of evidence (i) to constrain the ancient silicon cycle and changes in dissolved silicate concentration through application of silicon isotope (δ30Si) proxies and other techniques, (ii) to assess paleocirculation changes through approaches such as fish-tooth neodymium isotopes, and (iii) to construct more sophisticated age models with refined sedimentation rate estimates, for example, through application of cyclostratigraphic approaches to achieve resolution on astronomical timescales.
All data generated in this study are included in the Supplement.
The supplement related to this article is available online at: https://doi.org/10.5194/cp-17-1937-2021-supplement.
JW, SMB, and DEP designed the study. JW, KB, BSW, and EM performed sampling and analyses. EM performed the statistical analysis. All authors participated in interpreting the data. JW prepared the paper with input from all co-authors.
The contact author has declared that neither they nor their co-authors have any competing interests.
Publisher's note: Copernicus Publications remains neutral with regard to jurisdictional claims in published maps and institutional affiliations.
Jakub Witkowski acknowledges the support from the National Science Center (Poland). Walter Hale, Holger Kuhlmann, and the IODP Bremen Core Repository staff are thanked for efficient handling of multiple sample requests. Annette Olivarez Lyle, Mitchell Lyle (who also provided feedback on an earlier version of this paper), and Dorota Burska are thanked for advice on using the alkaline leaching method. We are indebted to Julita Tomkowiak, Agnieszka Ławecka, Adrianna Szaruga, Adrianna Januszkiewicz, and Zofia Stachowska for assistance in sample treatment and spectrophotometric analyses. John Barron and Louisa Bradtmiller are thanked for their constructive reviews.
This research has been supported by Narodowe Centrum Nauki (grant no. 2014/13/B/ST10/02988).
This paper was edited by David Thornalley and reviewed by John Barron and Louisa Bradtmiller.
Abelson, M. and Erez, J.: The onset of modern-like Atlantic meridional overturning circulation at the Eocene-Oligocene transition: evidence, causes, and possible implications for global cooling, Geochem. Geophy. Geosy., 18, 2177–2199, https://doi.org/10.1002/2017GC006826, 2017.
Anagnostou, E., John, E. H., Edgar, K. M., Foster, G. L., Ridgwell, A., Inglis, G. N., Pancost, R. D., Lunt, D. J., and Pearson, P. N.: Changing atmospheric CO2 concentration was the primary driver of early Cenozoic climate, Nature, 533, 380–384, https://doi.org/10.1038/nature17423, 2016.
Aubry, M.-P.: From chronology to stratigraphy: interpreting the lower and middle Eocene stratigraphic record in the Atlantic Ocean, in: Geochronology, Time Scales, and Global Stratigraphic Correlation, edited by: Berggren, W. A., Kent, D. V., Aubry, M.-P., and Hardenbol, J., SEPM Special Publication, 54, 213–274, https://doi.org/10.2110/pec.95.04.0213, 1995.
Barron, J. A., Stickley, C. E., and Bukry, D.: Paleoceanographic, and paleoclimatic constraints on the global Eocene diatom and silicoflagellate record, Palaeogeogr. Palaeocl., 422, 85–100, https://doi.org/10.1016/j.palaeo.2015.01.015, 2015.
Batenburg, S. J., Voigt, S., Friedrich, O., Osborne, A. H., Bornemann, A., Klein, T., Péréz-Díaz, L., and Frank, M.: Major intensification of Atlantic overturning circulation at the onset of Paleogene greenhouse warmth, Nat. Commun., 9, 4954, https://doi.org/10.1038/s41467-018-07457-7, 2018.
Berger, W. H.: Biogenous Deep-Sea Sediments: Fractionation by Deep-Sea Circulation, Geol. Soc. Am. Bull., 81, 1385–1402, https://doi.org/10.1130/0016-7606(1970)81[1385:BDSFBD]2.0.CO;2, 1970.
Bertolino, M., Cattaneo-Vietti, R., Pansini, M., Santini, C., and Bavestrello, G.: Siliceous sponge spicule dissolution: In field experimental evidences from temperate and tropical waters, Estuar. Coast. Shelf S., 184, 46–53, https://doi.org/10.1016/j.ecss.2016.10.044, 2017.
Bohaty, S. M., Zachos, J. C., Florindo, F., and Delaney, M. L.: Coupled greenhouse warming and deep-sea acidification in the middle Eocene, Paleoceanography, 24, PA2207, https://doi.org/10.1029/2008PA001676, 2009.
Boyle, R., Romans, B. W., Tucholke, B. E., Norris, R. D., Swift, S. A., and Sexton, F.: Cenozoic North Atlantic deep circulation history recorded in contourite drifts, offshore Newfoundland, Canada, Mar. Geol., 385, 185–203, https://doi.org/10.1016/j.margeo.2016.12.014, 2017.
Borrelli, C., Cramer, B. S., and Katz, M. E.: Bipolar Atlantic deepwater circulation in the middle-late Eocene: effects of Southern Ocean gateway openings, Paleoceanography, 29, 308–327, https://doi.org/10.1002/2012PA002444, 2014.
Caves, J. K., Jost, A. B., Lau, K. V., and Maher, K.: Cenozoic carbon cycle imbalances and a variable weathering feedback, Earth Planet. Sc. Lett., 450, 152–163, https://doi.org/10.1016/j.epsl.2016.06.035, 2016.
Cermeño, P., Falkowski, P. G., Romero, O. E., Schaller, M. F., and Vallina, S. M.: Continental erosion and the Cenozoic rise of marine diatoms, P. Natl. Acad. Sci. USA, 112, 4239–4244, https://doi.org/10.1073/pnas.1412883112, 2015.
Cleveland, W. S., Grosse, E., and Shyu, W. M.: Local regression models, in: Statistical Models in S, edited by: Chambers, J. M. and Hastie, T. J., Chapman & Hall, London, United Kingdom, 309–376, 1992.
Conley, D. J., Frings, P. J., Fontorbe, G., Clymans, W., and Stadmark, J.: Biosilicification Drives a Decline of Dissolved Si in the Oceans through Geologic Time, Front. Mar. Sci., 4, 397, https://doi.org/10.3389/fmars.2017.00397, 2017.
Coxall, H. K., Huck, C. E., Huber, M., Lear, C. H., Legarda-Lisarri, A., O'Regan, M., Sliwińska, K. K., van de Flierdt, T., de Boer, A. M., Zachos, J. C., and Backman, J.: Export of nutrient rich Northern Component Water preceded early Oligocene Antarctic glaciation, Nat. Geosci., 11, 190–196, https://doi.org/10.1038/s41561-018-0069-9, 2018.
Cramer, B. S., Toggweiler, J. R., Wright, J. D., Katz, M. E., and Miller, K. G.: Ocean overturning since the Late Cretaceous: Inferences from a new benthic foraminiferal isotope compilation, Paleoceanography, 24, PA4216, https://doi.org/10.1029/2008PA001683, 2009.
Cramwinckel, M. J., Huber, M., Kocken, I. J., Agnini, C., Bijl, P. K., Bohaty, S. M., Frieling, J., Goldner, A., Hilgen, F. J., Kip, E. L., Peterse, F., van der Ploeg, R., Röhl, U., Schouten, S., and Sluijs, A.: Synchronous tropical and polar temperature evolution in the Eocene, Nature, 559, 382–386, https://doi.org/10.1038/s41586-018-0272-2, 2018.
D'haenens, S., Bornemann, A., Claeys, P., Roöhl, U., Steurbaut, E., and Speijer, R. P.: A transient deep-sea circulation switch during Eocene Thermal Maximum 2, Paleoceanography, 29, 370–388, https://doi.org/10.1002/2013PA002567, 2014.
DeMaster, D. J.: The diagenesis of biogenic silica: chemical transformations occurring in the water column, seabed, and crust, in: Treatise on Geochemistry, 2nd edn., edited by: Holland, H. D. and Turekian, K. K., Elsevier, Amsterdam, The Netherlands, 9, 103–111, https://doi.org/10.1016/B978-0-08-095975-7.00704-X, 2014.
Diekmann, B., Kuhn, G., Gersonde, R., and Mackensen, A.: Middle Eocene to early Miocene environmental changes in the sub-Antarctic Southern Ocean: evidence from biogenic and terrigenous depositional patterns at ODP Site 1090, Global Planet. Change, 40, 295–313, https://doi.org/10.1016/j.gloplacha.2003.09.001, 2004.
Diester-Haass, L.: Middle Eocene to early Oligocene paleoceanography of the Antarctic Ocean (Maud Rise,ODP Leg 13, Site 689): change from a low to a high productivity ocean, Palaeogeogr. Palaeocl., 113, 311–334, https://doi.org/10.1016/0031-0182(95)00067-V, 1995.
Egan, K. E., Rickaby, R. E. M., Hendry, K. R., and Halliday, A. N.: Opening the gateways for diatoms primes Earth for Antarctic glaciation, Earth Planet. Sc. Lett., 375, 34-4-3, https://doi.org/10.1016/j.epsl.2013.04.030, 2013.
Fenner, J.: Middle Eocene to Oligocene planktonic diatom stratigraphy from deep sea drilling sites in the South Atlantic, Equatorial Pacific, and Indian oceans, Init. Repts DSDP, 75, 1245–1271, https://doi.org/10.2973/dsdp.proc.75.149.1984, 1984.
Fenner, J.: Taxonomy, stratigraphy, and paleoceanographic implications of Paleocene diatoms, Proc. ODP, Sci. Res., 114, 123–154, https://doi.org/10.2973/odp.proc.sr.114.137.1991, 1991.
Fiedler, P. C., Philbrick, V., and Chavez, F. P.: Oceanic upwelling and productivity in the eastern tropical Pacific, Limnol. Oceanogr., 36, 1834–1850, https://doi.org/10.4319/lo.1991.36.8.1834, 1991.
Fontorbe, G., Frings, P. J., De La Rocha, C. L., Hendry, K. R., and Conley, D. J.: A silicon depleted North Atlantic since the Palaeogene: evidence from sponge and radiolarian silicon isotopes, Earth Planet. Sc. Lett., 453, 67–77, https://doi.org/10.1016/j.epsl.2016.08.006, 2016.
Fontorbe, G., Frings, P. J., De La Rocha, C. L., Hendry, K. R., and Conley, D. J.: Constraints on Earth system functioning at the Paleocene-Eocene Thermal Maximum from the marine silicon cycle, Paleoceanography and Paleoclimatology, 35, e2020PA003873, https://doi.org/10.1029/2020PA003873, 2020.
Foster, G., Royer, D., and Lunt, D., Future climate forcing potentially without precedent in the last 420 million years, Nat. Commun., 8, 14845, https://doi.org/10.1038/ncomms14845, 2017.
Frings, P.: Revisiting the dissolution of biogenic Si in marine sediments: a key term in the ocean Si budget, Acta Geochimica, 36, 429–432, https://doi.org/10.1007/s11631-017-0183-1, 2017.
Froelich, F. and Misra, S.: Was the late Paleocene-early Eocene hot because Earth was flat? An ocean lithium isotope view of mountain building, continental weathering, carbon dioxide, and Earth's Cenozoic climate, Oceanography, 27, 36–49, https://doi.org/10.5670/oceanog.2014.06, 2015.
Gombos Jr., A. M.: Early and Middle Eocene diatom evolutionary events, Bacillaria, 5, 225–243, 1982.
Gradstein, F. M. and Sheridan, R. E.: On the Jurassic Atlantic Ocean and a synthesis of results of DSDP Project Leg 76: Init. Repts DSDP, 76, 913–943, https://doi.org/10.2973/dsdp.proc.76.144.1983, 1983.
Gradstein, F. M., Ogg, J. G., Schmitz, M. D., and Ogg, G. M. (Eds.): The Geologic Time Scale 2012, Elsevier, Amsterdam, The Netherlands, 2, 1144 pp., https://doi.org/10.1016/C2011-1-08249-8, 2012.
Gula, J., Molemaker, M. J., and McWilliams, J. C.: Gulf Stream Dynamics along the Southeastern U.S. Seaboard, J. Phys. Oceanogr., 45, 690–715, https://doi.org/10.1175/JPO-D-14-0154.1, 2015.
Gula, J., Molemaker, M. J., and McWilliams, J. C.: Submesoscale Dynamics of a Gulf Stream Frontal Eddy in the South Atlantic Bight, J. Phys. Oceanogr., 46, 305–325, https://doi.org/10.1175/JPO-D-14-0258.1, 2016.
Handoh, I. C., Bigg, G. R., and Jones, E. J. W.: Evolution of upwelling in the Atlantic Ocean basin, Palaeogeogr. Palaeocl., 202, 31–58, https://doi.org/10.1016/S0031-0182(03)00571-6, 2003.
Hayes, C. T., Costa, K. M., Anderson, R. F., Calvo, E., Chase, Z., Demina, L. L., Dutay, J.-C., German, C. R., Heimbürger-Boavida, L.-E., Jaccard, S. L., Jacobel, A., Kohfeld, K. E., Kravchishina, M. D., Lippold, J., Mekik, F., Missiaen, L., Pavia, F. J., Paytan, A., Pedrosa-Pamies, R., Petrova, M. V., Rahman, S., Robinson, L. F., Roy-Barman, M., Sanchez-Vidal, A., Shiller, A., Tagliabue, A., Tessin, A. C., van Hulten, M., and Zhang, J.: Global ocean sediment composition and burial flux in the deep sea, Global Biogeochem. Cy., 35, e2020GB006769, https://doi.org/10.1029/2020GB006769, 2020.
Hendry, K. R., Marron, A. O., Vincent, F., Conley, D. J., Gehlen, M., Ibarbalz, F. M., Quéguiner, B., and Bowler, C.: Competition between silicifiers and non-silicifiers in the past and present ocean and its evolutionary impacts, Front. Mar. Sci., 5, 22, https://doi.org/10.3389/fmars.2018.00022, 2018.
Henehan, M. J., Edgar, K. M., Foster, G. L., Penman, D. E., Hull, P. M., Greenop, R., Anagnostou, E., and Pearson, P. N.: Revisiting the Middle Eocene Climatic Optimum “Carbon Cycle Conundrum” with new estimates of atmospheric pCO2 from boron isotopes, Paleoceanography and Paleoclimatology, 35, e2019PA003713, https://doi.org/10.1029/2019PA003713, 2020.
Hilting, A. K., Kump, L. R., and Bralower, T. J.: Variations in the oceanic vertical carbon isotope gradient and their implications for the Paleocene-Eocene biological pump, Paleoceanography, 23, PA3222, https://doi.org/10.1029/2007PA001458, 2008.
Hohbein, M. W., Sexton, P. F., and Cartwright, J. A.: Onset of North Atlantic Deep Water production coincident with inception of the Cenozoic global cooling trend, Geology, 40, 255–258, https://doi.org/10.1130/G32461.1, 2012.
Hollis, C. J.: Data report: siliceous microfossil abundance in IODP Expedition 342 sediments, Proc. IODP, 342, 1–16, https://doi.org/10.2204/iodp.proc.342.201.2017, 2014.
Iwasaki, S., Takahashi, K., Ogawa, Y., Uehara, S., and Vogt, C.: Alkaline leaching characteristics of biogenic opal in Eocene sediments from the central Arctic Ocean: a case study in the ACEX cores, J. Oceanogr., 70, 241–249, https://doi.org/10.1007/s10872-014-0227-7, 2014.
Kamikuri, S.-I. and Wade, B. S.: Radiolarian magnetobiochronology and faunal turnover across the middle/late Eocene boundary at Ocean Drilling Program Site 1052 in the western North Atlantic Ocean, Mar. Micropaleontol., 88–89, 41–53, https://doi.org/10.1016/j.marmicro.2012.03.001, 2012.
Kasting, J. F.: The Goldilocks Planet? How Silicate Weathering Maintains Earth “Just Right”, Elements, 15, 235–240, https://doi.org/10.2138/gselements.15.4.235, 2019.
Katz, M. E., Cramer, B. S., Toggweiler, J. R., Esmay, G., Liu, Ch., Miller, K. G., Rosenthal, Y., Wade, B. S., and Wright, J. D.: Impact of Antarctic Circumpolar Current Development on Late Paleogene Ocean Structure, Science, 332, 1076–1079, https://doi.org/10.1126/science.1202122, 2011.
Kirtland-Turner, S., Sexton, P. F., Charles, C. D., and Norris, R. D.: Persistence of carbon release events through the peak of early Eocene global warmth, Nat. Geosci., 7, 748–751, https://doi.org/10.1038/ngeo2240, 2014.
Klemm, V., Levasseur, S., Frank, M., Hein, J. R., and Halliday, A. N.: Osmium isotope stratigraphy of a marine ferromanganese crust, Earth Planet. Sc. Lett., 238, 42–48, https://doi.org/10.1016/j.epsl.2005.07.016, 2005.
Lazarus, D., Barron, J. A., Renaudie, J., Diver, P., and Türke, A.: Cenozoic Planktonic Marine Diatom Diversity and Correlation to Climate Change, PLOS ONE, 9, e84857, https://doi.org/10.1371/journal.pone.0084857, 2014.
Lee, T. N., Yoder, J. A., and Atkinson, L. P.: Gulf Stream Frontal Eddy Influence on Productivity of the Southeast U.S. Continental Shelf, J. Geophys. Res., 96, 22191–22205, https://doi.org/10.1029/91JC02450, 1991.
Lisitzin, A. P.: Distribution of siliceous microfossils in suspension and in bottom sediments, in: The Micropalaeontology of Oceans, edited by: Funnell, B. M. and Riedel, W. R., Cambridge University Press, Cambridge, United Kingdom, 173–196, 1971.
Lyle, M., Olivarez Lyle, A., Backman, J., and Tripati, A.: Biogenic Sedimentation in the Eocene Equatorial Pacific—The Stuttering Greenhouse and Eocene Carbonate Compensation Depth, Proc. ODP, Sci. Res., 199, 1–35, https://doi.org/10.2973/odp.proc.sr.199.219.2005, 2005.
Maldonado, M., Camona, M. C., Uriz, M. J., and Cruzado, A.: Decline in Mesozoic reef-building sponges explained by silicon limitation, Nature, 401, 785–788, https://doi.org/10.1038/44560, 1999.
Malviya, S., Scalco, E., Audic, S., Vincent, F., Veluchamy, A., Poulain, J., Wincker, P., Iudicone, D., de Vargas, C., Bittner, L., Zingone, A., and Bowler, C.: Insights into global diatom distribution and diversity in the world's ocean, P. Natl. Acad. Sci. USA, 113, 1516–1525, https://doi.org/10.1073/pnas.1509523113, 2016.
Miller, K. G., Browning, J. V., Schmelz, W. J., Kopp, R. E., Mountain, G. S., and Wright, J. D.: Cenozoic sea-level and cryospheric evolution from deep-sea geochemical and concinental margin records, Sci. Adv., 6, eaaz1346, https://doi.org/10.1126/sciadv.aaz1346, 2020.
Miskell, K. J., Brass, G. W., and Harrison, C. G. A.: Global patterns in opal deposition from Late Cretaceous to Late Miocene, AAPG Bull., 69, 996–1012, https://doi.org/10.1306/AD462B41-16F7-11D7-8645000102C1865D, 1985.
Misra, S. and Froelich, N.: Lithium isotope history of Cenozoic seawater: changes in silicate weathering and reverse weathering, Science, 335, 818–823, https://doi.org/10.1126/science.1214697, 2012.
Montes, C., Cardona, A., McFadden, R., Morón, S. E., Silva, C. A., Restrepo-Moreno, S., Ramírez, D. A., Hoyos, N., Wilson, J., Farris, D., Bayona, G. A., Jaramillo, C. A., Valencia, V., Bryan, J., and Flores, J. A.: Evidence for middle Eocene and younger land emergence in central Panama: Implications for Isthmus closure, Geol. Soc. Am. Bull., 124, 780–799, https://doi.org/10.1130/B30528.1, 2012.
Moore Jr., T. C.: Radiolaria: change in skeletal weight and resistance to solution, Geol. Soc. Am. Bull., 80, 2103–2108, https://doi.org/10.1130/0016-7606(1969)80[2103:RCISWA]2.0.CO;2, 1969.
Moore Jr., T. C., Jarrard, R. D., Olivarez Lyle, A., and Lyle, M.: Eocene biogenic silica accumulation rates at the Pacific equatorial divergence zone, Paleoceanography, 23, PA220, https://doi.org/10.1029/2007PA001514, 2008.
Muttoni, G. and Kent, D. V.: Widespread formation of cherts during the early Eocene climate optimum, Palaeogeogr. Palaeocl., 253, 348–362, https://doi.org/10.1016/j.palaeo.2007.06.008, 2007.
Newsam, C., Bown, R., Wade, B. S., and Jones, H. L.: Muted calcareous nannoplankton response at the Middle/Late Eocene Turnover event in the western North Atlantic Ocean, Newsl. Stratig., 50, 297–309, https://doi.org/10.1127/nos/2016/0306, 2017.
Nielsen, S. G., Mar-Gerrison, S., Gannoun, A., LaRowe, D., Klemm, V., Halliday, A. N., Burton, K. W., and Hein, J. R.: Thallium isotope evidence for a permanent increase in marine organic carbon export in the early Eocene, Earth Planet. Sc. Lett., 278, 397–307, https://doi.org/10.1016/j.epsl.2008.12.010, 2009.
Nishimura, A.: Paleocene radiolarian biostratigraphy in the northwest Atlantic at Site 384, Leg 43, of the Deep Sea Drilling Project, Micropaleontology, 38, 317–362, 1992.
Ogg, J. G. and Bardot, L.: Aptian through Eocene magnetostratigraphic correlation of the Blake Nose Transect (Leg 171B), Florida Continental Margin, Proc. ODP, Sci. Res., 171B, 1–58, https://doi.org/10.2973/odp.proc.sr.171B.104.2001, 2001.
Ocean Drilling Stratigraphic Network: https://www.odsn.de/, last access: 15 April 2021.
Olivarez Lyle, A. and Lyle, M.: Determination of biogenic opal in pelagic marine sediments: a simple method revisited, Proc. ODP, Init. Repts., 199, 1–21, https://doi.org/10.2973/odp.proc.ir.199.106.2002, 2002.
Oreshkina, T. V. and Aleksandrova, G. N.: Terminal paleocene of the Volga middle reaches: Biostratigraphy and paleosettings, Stratigr. Geol. Correl., 15, 206–230, https://doi.org/10.1134/S0869593807020062, 2007.
Pelegrí, J. L., Csanady, G. T., and Martins, A.: The North Atlantic Nutrient Stream, J. Oceanogr., 52, 275–299, https://doi.org/10.1007/BF02235924, 1996.
Penman, D. E.: Silicate weathering and North Atlantic silica burial during the Paleocene-Eocene Thermal Maximum, Geology, 44, 731–734, https://doi.org/10.1130/G37704.1, 2016.
Penman, D. E., Keller, A., D'haenens, S., Turner, S. K., and Hull, P. M.: Atlantic Deep-Sea Cherts Associated With Eocene Hyperthermal Events, Paleoceanography and Paleoclimatology, 34, 287–299, https://doi.org/10.1029/2018PA003503, 2019.
Penman, D. E., Caves Rugenstein, J. K., Ibarra, D. E., and Winnick, M.J .: Silicate weathering as a feedback and forcing in Earth's climate and carbon cycle, Earth-Sci. Rev., 209, 103298, https://doi.org/10.1016/j.earscirev.2020.103298, 2020.
Piela, C., Lyle, M., Marcantonio, F., Baldauf, J., and Olivarez Lyle, A.: Biogenic sedimentation in the equatorial Pacific: Carbon cycling and paleoproduction, 12–24 Ma, Paleoceanography, 27, PA2204, https://doi.org/10.1029/2011PA002236, 2012.
Pinet, P. R., Popenoe, P., and Nelligan, D. F.: Gulf Stream: Reconstruction of Cenozoic flow patterns over the Blake Plateau, Geology, 9, 266–270, https://doi.org/10.1130/0091-7613(1981)9<266:GSROCF>2.0.CO;2, 1981.
Ragueneau, O., Tréguer, P., Leynaert, A., Anderson, R. F., Brzezinski, M. A., DeMaster, D. J., Dugdale, R. C., Dymond, J., Fischer, G., François, R., Heinze, C., Maier-Reimer, E., Martin-Jézéquel, V., Nelson, D. M., and Quéquiner, B.: A review of the Si cycle in the modern ocean: recent progress and missing gaps in the application of biogenic opal as a paleoproductivity proxy, Global Planet. Change, 26, 317–365, https://doi.org/10.1016/S0921-8181(00)00052-7, 2000.
Ravizza, G. and Peucker-Ehrenbrink, B.: The marine record of the Eocene–Oligocene transition: the interplay of weathering and glaciation, Earth Planet. Sc. Lett., 210, 151–165, https://doi.org/10.1016/S0012-821X(03)00137-7, 2003.
Ravizza, G., Norris, R. N., Blusztajn, J., and Aubry, M.-P.: An osmium isotope excursion associated with the Late Paleocene thermal maximum: Evidence of intensified chemical weathering, Paleoceanography, 16, 155–163, https://doi.org/10.1029/2000PA000541, 2001.
Renaudie, J.: Quantifying the Cenozoic marine diatom deposition history: links to the C and Si cycles, Biogeosciences, 13, 6003–6014, https://doi.org/10.5194/bg-13-6003-2016, 2016.
Richardson, P. L.: Florida Current, Gulf Stream, and Labrador Current, in: Encyclopedia of Ocean Sciences, edited by: Steele, J. H., Elsevier, Amsterdam, The Netherlands, 2, 1054–1064, https://doi.org/10.1006/rwos.2001.0357, 2001.
Roughan, M., Keating, S. R., Schaeffer, A., Heredia, C. P., Rocha, C., Griffin, D., Robertson, R., and Suthers, I. M.: A tale of two eddies: The biophysical characteristics of two contrasting cyclonic eddies in the East Australian Current System, J. Geophys. Res.-Oceans, 122, 2494–2518, https://doi.org/10.1002/2016JC012241, 2017.
Röhl, U., Norris, R. D., and Ogg, J. G.: Cyclostratigraphy of upper Paleocene and lower Eocene sediments at Blake Nose Site 1051 (western North Atlantic), Geol. Soc. Am. Spec. Pap., 369, 567–589, https://doi.org/10.1130/0-8137-2369-8.567, 2003.
Salamy, K. A. and Zachos, J. C.: Latest Eocene-early Oligocene climate change and Southern Ocean fertility: inferences from sediment accumulation and stable isotope data, Palaeogeogr. Palaeocl., 145, 61–77, https://doi.org/10.1016/S0031-0182(98)00093-5, 1999.
Sanchez-Franks, A. and Zhang, R.: Impact of the Atlantic meridional overturning circulation on the decadal variability of the Gulf Stream path and regional chlorophyll and nutrient concentrations, Geophys. Res. Lett., 42, 9889–9897, https://doi.org/10.1002/2015GL066262, 2015.
Shipboard Scientific Party: Introduction, Proc. ODP, Init. Repts., 171B, 5–10, https://doi.org/10.2973/odp.proc.ir.171B.101.1998, 1998a.
Shipboard Scientific Party: Site 1049, Proc. ODP, Init. Repts., 171B, 47–91, https://doi.org/10.2973/odp.proc.ir.171B.103.1998, 1998b.
Shipboard Scientific Party: Site 1050, Proc. ODP, Init. Repts., 171B, 93–170, https://doi.org/10.2973/odp.proc.ir.171B.104.1998, 1998c.
Shipboard Scientific Party: Site 1051, Proc. ODP, Init. Repts., 171B, 171–239, https://doi.org/10.2973/odp.proc.ir.171B.105.1998, 1998d.
Shipboard Scientific Party: Site 1052, Proc. ODP, Init. Repts., 171B, 241–319, https://doi.org/10.2973/odp.proc.ir.171B.106.1998, 1998e.
Shipboard Scientific Party: Site 1053, Proc. ODP, Init. Repts., 171B, 321–348, https://doi.org/10.2973/odp.proc.ir.171B.107.1998, 1998f.
Sims, P. A., Mann, D. G., and Medlin, L. K.: Evolution of the diatoms: insights from fossil, biological and molecular data, Phycologia, 45, 361–402, https://doi.org/10.2216/05-22.1, 2006.
Sluijs, A., Zeebe, R. E., Bijl, P. K., and Bohaty, S. M.: A middle Eocene carbon cycle conundrum: Nat. Geosci., 6, 429–434, https://doi.org/10.1038/ngeo1807, 2013.
Smetacek, V.: Diatoms and the ocean carbon cycle: Protist, 150, 25–32, https://doi.org/10.1016/S1434-4610(99)70006-4, 1999.
Thomas, D. J., Bralower, T. J., and Jones, C. E.: Neodymium isotopic reconstruction of late Paleocene-early Eocene thermohaline circulation, Earth Planet. Sc. Lett., 209, 309–322, https://doi.org/10.1016/S0012-821X(03)00096-7, 2003.
Tréguer, P. J. and De La Rocha, C. L.: The World Ocean silica cycle, Annu. Rev. Mar. Sci., 5, 477–501, https://doi.org/10.1146/annurev-marine-121211-172346, 2013.
Van Cappellen, P., Dixit, S., and van Beusekom, J.: Biogenic silica dissolution in the oceans: Reconciling experimental and field-based dissolution rates, Global Biogeochem. Cy., 16, 1075, https://doi.org/10.1029/2001GB001431, 2002.
van der Ploeg, R., Selby, D., Cramwinckel, M. J., Li, Y., Bohaty, S. M., Middelburg, J. J., and Sluijs, A.: Middle Eocene greenhouse warming facilitated by diminished weathering feedback, Nat. Commun., 9, 2877, https://doi.org/10.1038/s41467-018-05104-9, 2018.
Vahlenkamp, M., Niezgodzki, I., De Vleeschouwer, D., Lohmann, G., Bickert, T., and Pälike, H.: Ocean and climate response to North Atlantic seaway changes at the onset of long-term Eocene cooling, Earth Planet. Sc. Lett., 498, 185–195, https://doi.org/10.1016/j.epsl.2018.06.031, 2018.
Via, R. K. and Thomas, D. J.: Evolution of Atlantic thermohaline circulation: early Oligocene onset of deep-water production in the North Atlantic, Geology, 34, 441–444, https://doi.org/10.1130/G22545.1, 2006.
Wade, B. S. and Kroon, D.: Middle Eocene regional climate instability: Evidence from the western North Atlantic, Geology, 30, 1011–1014, https://doi.org/10.1130/0091-7613(2002)030<1011:MERCIE>2.0.CO;2, 2002.
Wade, B. S., Fucek, V. P., Kamikuri, S.-I., Bartol, M., Luciani, V., and Pearson, P. N.: Successive extinctions of muricate planktonic foraminifera (Morozovelloides and Acarinina) as a candidate for marking the base Priabonian, Newsl. Stratig., 45, 245–262, https://doi.org/10.1127/0078-0421/2012/0023, 2012.
Wade, B. S., O'Neill, J. F., Phujareanchaiwon, C., Ali, I., Lyle, M., and Witkowski, J.: Evolution of deep-sea sediments across the Paleocene-Eocene and Eocene-Oligocene boundaries, Earth-Sci. Rev., 211, 103403, https://doi.org/10.1016/j.earscirev.2020.103403, 2020.
Walker, J. C., Hays, B., and Kasting, J. F.: A negative feedback mechanism for the long-term stabilization of Earth's surface temperature, J. Geophys. Res., 86, 9776–9782, https://doi.org/10.1029/JC086iC10p09776, 1981.
Warnock, J. P. and Scherer, R. P.: Diatom species abundance and morphologically-based dissolution proxies in coastal Southern Ocean assemblages, Cont. Shelf Res., 102, 1–8, https://doi.org/10.1016/j.csr.2015.04.012, 2015.
Westerhold, T., Röhl, U., Donner, B., and Zachos, J. C.: Global extent of early Eocene hyperthermal events: A new Pacific benthic foraminiferal isotope record from Shatsky Rise (ODP Site 1209), Paleoceanography and Paleoclimatology, 33, 626–642, https://doi.org/10.1029/2017PA003306, 2018a.
Westerhold, T., Röhl, U., Donner, B., Friederichs, T., Kordesch, W. E. C., Bohaty, S. M., Hodell, D. A., Laskar, J., and Zeebe, R. E.: Late Lutetian Thermal Maximum – crossing a thermal threshold in Earth's climate system?, Geochem. Geophys. Geosy., 19, 73–82, https://doi.org/10.1002/2017GC007240, 2018b.
Witkowski, J., Bohaty, S. M., Edgar, K. M., and Harwood, D. M.: Rapid fluctuations in mid-latitude siliceous plankton production during the Middle Eocene Climatic Optimum (ODP Site 1051, western North Atlantic), Mar. Micropaleontol., 106, 110–129, https://doi.org/10.1016/j.marmicro.2014.01.001, 2014.
Witkowski, J., Harwood, D. M., Wade, B. S., and Bryłka, K.: Rethinking the chronology of early Paleogene sediments in the western North Atlantic using diatom biostratigraphy, Mar. Geol., 424, 106168, https://doi.org/10.1016/j.margeo.2020.106168, 2020a.
Witkowski, J., Penman, D., Bryłka, K., Wade, B. S., Matting, S., Harwood, D. M., and Bohaty, S. M.: Early Paleogene biosiliceous sedimentation in the Atlantic Ocean: testing the inorganic origin hypothesis for Paleocene and Eocene chert and porcellanite, Palaeogeogr. Palaeocl., 556, 109896, https://doi.org/10.1016/j.palaeo.2020.109896, 2020b.
Yool, A. and Tyrrell, T.: Role of diatoms in regulating the ocean's silicon cycle, Global Biogeochem. Cy., 17, 1103, https://doi.org/10.1029/2002GB002018, 2003.
Yool, A. and Tyrrell, T.: Implications for the history of Cenozoic opal deposition from a quantitative model, Palaeogeogr. Palaeocl., 218, 239–255, https://doi.org/10.1016/j.palaeo.2004.12.017, 2005.
Zachos, J. C., Quinn, T. M., and Salamy, K. A.: High-resolution (104 years) deep-sea foraminiferal stable isotope records of the Eocene-Oligocene climate transition, Paleoceanography, 11, 251–266, https://doi.org/10.1029/96PA00571, 1996.
Zachos, J. C., Pagani, M., Sloan, L., Thomas, E., and Billups, K.: Trends, rhythms, and aberrations in global climate 65 Ma to present, Science, 292, 686–693, https://doi.org/10.1126/science.1059412, 2001.
Zachos, J. C., Dickens, G. R., and Zeebe, R. E.: An early Cenozoic perspective on greenhouse warming and carbon-cycle dynamics, Nature, 451, 279–283, https://doi.org/10.1038/nature06588, 2008.