the Creative Commons Attribution 4.0 License.
the Creative Commons Attribution 4.0 License.
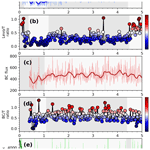
Five thousand years of fire history in the high North Atlantic region: natural variability and ancient human forcing
Delia Segato
Maria Del Carmen Villoslada Hidalgo
Ross Edwards
Elena Barbaro
Paul Vallelonga
Helle Astrid Kjær
Marius Simonsen
Bo Vinther
Niccolò Maffezzoli
Roberta Zangrando
Clara Turetta
Dario Battistel
Orri Vésteinsson
Carlo Barbante
Biomass burning influences global atmospheric chemistry by releasing greenhouse gases and climate-forcing aerosols. There is controversy about the magnitude and timing of Holocene changes in biomass burning emissions from millennial to centennial timescales and, in particular, about the possible impact of ancient civilizations. Here we present a 5 kyr record of fire activity proxies levoglucosan, black carbon, and ammonium measured in the RECAP (Renland ice cap) ice core, drilled in coastal eastern Greenland, and therefore affected by processes occurring in the high North Atlantic region. Levoglucosan and ammonium fluxes are high from 5 to 4.5 kyr BP (thousand years before 2000 CE) followed by an abrupt decline, possibly due to monotonic decline in Northern Hemisphere summer insolation. Levoglucosan and black carbon show an abrupt decline at 1.1 kyr BP, suggesting a decline in the wildfire regime in Iceland due to the extensive land clearing caused by Viking colonizers. All fire proxies reach a minimum during the second half of the last century, after which levoglucosan and ammonium fluxes increase again, in particular over the last 200 years. We find that the fire regime reconstructed from RECAP fluxes seems mainly related to climatic changes; however over the last millennium human activities might have influenced wildfire frequency/occurrence substantially.
- Article
(3186 KB) - Full-text XML
-
Supplement
(1436 KB) - BibTeX
- EndNote
Extensive wildfires, also at high latitudes, have recently generated worldwide attention and raised concerns about the impacts of humans and climate change on fire regimes. During summer 2020 wildfires over the Arctic Circle emitted 35 % more CO2 than the previous year, with a significant contributor being peatland fires (Witze, 2020). However, little is known about the patterns and driving forces of fire activity in the past. Quantitative observations of wildfires are severely limited both in time and space, and coverage in global datasets based on satellite observations began in 1974 (Chuvieco et al., 2019). In order to understand the climate–human–fire relationship over long timescales, proxy records of biomass burning are invaluable.
Fire is influenced by human activities, vegetation, and climate, and it is a key Earth system process (Bowman et al., 2009; Keywood et al., 2013). As a major component of the carbon cycle, fire interacts with the climate system by releasing particulates and greenhouse gases, including CO2, CO, CH4, NOx, and black carbon (Bowman et al., 2009). Climatic conditions (temperature, insolation changes, atmospheric CO2, precipitation) are the fundamental drivers for the ignition and spread of fire (Andela et al., 2017; Marlon et al., 2008, 2013; Power et al., 2008; Molinari et al., 2018). Fuel load and vegetation type also influence fire behaviour, with high levels of biomass burning/fire severity coinciding with the dominance of fire invaders and lower biomass burning/severity coinciding with the dominance of resisters (Feurdean et al., 2020a). Understanding the causes and consequences of fires is critical for assessing the state of the Earth system, because of the close relationship between fire, vegetation, and climate.
Anthropogenic activities have influenced the environment well before the Industrial Revolution, as several studies suggest (Ruddiman, 2003; Doughty, 2013). All the continents (except Antarctica) were settled by the beginning of the Holocene; thus this period provides crucial context for fire–human–climate interactions and an opportunity to disentangle climate and human contributions in changing fire regimes (Marlon et al., 2013). It has been argued that human activities have influenced fire activity for millennia (Marlon et al., 2008; Power et al., 2008). In the earliest phase of agricultural development, farmers used fire to clear land through the slash-and-burn technique (Ruddiman and Ellis, 2009). With the establishment of the first agricultural societies in Europe in the mid-Holocene, humans substantially altered the European landscape (Price, 2000). The attribution of changes in fire regimes to human impact is largely based on the synchronicity of these changes and indicators of human activity, such as changes in erosion rates, vegetation, and land use (Marlon et al., 2013). Ruddiman (2003) explicitly argued that land use changes in Eurasia during the early to mid-Holocene could explain increases in CH4 and CO2 atmospheric concentrations and that these changes had a significant impact on climate. Fire variability inferred from the NEEM (North Greenland Eemian) ice core (Greenland) was associated with droughts as well as temperature and summer insolation variability; however from 4 kyr BP (thousand years before 2000 CE) fire trends could not be explained without considering human influence in altering vegetation distribution, especially in Europe (Zennaro et al., 2015). Sapart et al. (2012) found an increase in pyrogenic CH4 emissions at the times of the Roman Empire (2.1–1.7 kyr BP), the Medieval Climate Anomaly (1.2–0.8 kyr BP), and the Little Ice Age (0.7–0.4 kyr BP) from the analysis of CH4 isotopic composition of the air trapped in the Greenlandic EUROCORE and NEEM ice cores. The pyrogenic CH4 increase associated with the Roman Empire was attributed to an increase of charcoal use from metal production and to the contemporary civilizations in China and India. On the other hand, the increase during medieval times has been associated with both extended droughts in northern Europe and accelerating deforestation in both Europe and Asia, while the increase detected during the Little Ice Age has been explained by natural wildfires and by rapid land clearance in the Northern Hemisphere (NH) (Sapart et al., 2012).
A diverse range of palaeo-tracers has been used to reconstruct biomass burning from different climate archives such as fire scars on tree rings, ice core records of gases and aerosol-borne chemicals (Legrand et al., 2016; Rubino et al., 2015), and sedimentary charcoal records (Marlon et al., 2008, 2016; Power et al., 2008). These records reflect a wide range of different regions of fire locations, frequency, distribution, and intensity integrated over a wide span of temporal scales (Grieman et al., 2018; Battistel et al., 2018; Lim et al., 2017). Since charcoal particles settle rapidly, one record is only locally representative (in the order of tens of kilometres) and several charcoal records need to be assembled in order to obtain a regional reconstruction of fire activity (Marlon et al., 2008; Blarquez et al., 2014). Furthermore, several regions, like Siberia, are under-represented in the available charcoal reconstructions, reducing their potential in the reconstruction of fire history over wide spatial areas.
Ice cores from polar regions, on the other hand, are extensively used to reconstruct past climate conditions and can provide fire records (Legrand et al., 2016). Despite being geographical point measurements, ice cores represent a record of air, moisture, and aerosols sourced from regional or even hemispheric scales with up to annual and sub-annual temporal resolution (Zennaro et al., 2015; Legrand et al., 2016; Simonsen et al., 2019). They also have the great advantage of catching rapid events such as volcanic eruptions and wildfires.
Historically, the ice concentrations of some impurities like ammonium (NH) and K+ were suggested to be partially influenced by forest fire emissions. However, these compounds are not specific proxies as their background variations also reflect continuous biogenic emissions from vegetation and soils, while only peak values can be associated with biomass burning events (Fischer et al., 2015). K+, additionally, has been found to be highly sensitive to contamination, making it difficult to measure its species in ice (Legrand et al., 2016). Recently, however, most of the attention has been given to two specific fire proxies, levoglucosan and black carbon (BC), the latter being a specific tracer of biomass burning in pre-industrial times (Osmont et al., 2019). Despite NH being influenced by biogenic emissions from ice-free areas in Greenland, it has been included in this study for comparison.
Levoglucosan (1,6-anhydro-β-D-glucopyranose) is the most abundant monosaccharide anhydride released when cellulose combustion occurs at temperatures >300 ∘C (Simoneit, 1999). It is injected in the atmosphere in convective smoke plumes and deposited on glacier surfaces through wet and dry deposition (Gambaro et al., 2008) with a residence time of up to 2 weeks (Bhattarai et al., 2019). Since levoglucosan is strongly water-soluble, it may be leached during the melt–refreeze process, reshaping the post-depositional distribution (You et al., 2016). Due to its high emission factors and relatively high concentrations in the ambient aerosols, it is an ideal marker compound for biomass burning (Hoffmann et al., 2010).
BC is the light-absorbing refractory carbonaceous matter emitted during incomplete combustion of fossil and biofuels in fires ignited by both natural and human sources (McConnell et al., 2007). BC is an important indicator of biomass burning for palaeoclimate reconstructions, as it is a specific fire proxy for pre-industrial times and can be measured at high resolution with continuous-flow analysis (CFA; McConnell et al., 2007). BC does not refer to a single well-defined compound because carbonaceous aerosols are emitted in the form of a continuum of compounds with different physical and chemical properties. BC has very low chemical reactivity in the atmosphere, and its residence time is about 1 week; its primary removal process is wet deposition, with dry deposition contributing 15 %–40 % of the total removal (Bond et al., 2013; Cape et al., 2012; Barrett et al., 2019).
Previous studies found levoglucosan and BC to have similar trends in Greenland ice cores (Legrand et al., 2016), giving a further hint that ice core archives provide a complementary tool to charcoal records in examining the link between climate, human influence, and fire activity. By now, several records of fire proxies from Greenland ice cores have reconstructed fire history for the last few centuries; however very few extend over the past millennia. In this work we present 5 kyr long records of levoglucosan, BC, and NH from the Renland ice core (71∘18′18′′ N, 26∘43′24′′ W). The main objective of this paper is to elucidate the role of climate and human activities in fire activity in the almost unstudied area of the high North Atlantic before the industrial era.
The RECAP (Renland ice cap) project retrieved in 2015 a 584 m ice core drilled to the bedrock on the Renland ice cap. The Renland ice cap is situated in eastern Greenland on a high-elevation plateau on the Renland peninsula in the Scoresby Sund fjord (71∘18′18′′ N, 26∘43′24′′ W). The ice cap is constrained by surrounding topography, and its eastern plateau reaches an elevation of 2340 m at its summit. Its coastal location provides important geographic climate information that can be compared with central Greenland ice cores as well as providing a sensitive indicator of changes at the margins of the Greenland Ice Sheet.
The core was stored frozen and shipped to Europe, where it was cut at AWI (Alfred Wegener Institute, Bremerhaven, Germany) and processed at the Centre for Ice and Climate (Niels Bohr Institute, University of Copenhagen, Denmark). The samples analysed for levoglucosan were collected discretely every 55 cm from a continuous ice core melting system (Bigler et al., 2011) as part of the CFA campaign conducted at the University of Copenhagen in autumn 2015 (Maffezzoli et al., 2019; Simonsen et al., 2019), while BC and NH were measured continuously. After collection, the discrete samples were immediately frozen at −20 ∘C and kept in the dark until analysis. In this work we consider the top 482 m of the core, corresponding to the last 5 kyr. The chronology is achieved by annual layer counting down to 458.3 m and below this point by volcanic matching to the GICC05 timescale (Simonsen et al., 2019).
2.1 Levoglucosan, BC, and NH analysis
Levoglucosan was determined using high-performance liquid chromatography/negative ion electrospray ionization–tandem mass spectrometry (HPLC/(-)ESI-MS/MS). This analytical method allows the direct injection of melted samples spiked with 13C6-labelled internal standard into the HPLC instrument, avoiding contamination during pre-analytical steps (Gambaro et al., 2008; Zennaro et al., 2014; Battistel et al., 2018). All pre-analytical steps were performed under a class 100 clean bench located in a class 100 clean room at Ca' Foscari University of Venice. The Purelab Ultra system (Elga, High Wycombe, UK) was used to produce the ultrapure water (18.2 MΩ cm, 0.01 TOC) utilized in all analytical and pre-analytical procedures (i.e. cleaning and decontamination procedures, standard solution preparation, and chromatographic analysis) (Gambaro et al., 2008).
BC analysis was conducted using a BC analyser (SP2, Droplet Measurement Technologies, Boulder, Colorado) connected to the CFA system, following the method of McConnell et al. (2007). The SP2 measures mass of individual BC particles using laser-induced incandescence. BC particles absorb sufficient energy as they pass through the laser beam and reach a temperature at which they incandesce. The intensity of the incandescence is measured with a photomultiplier tube and recorded. The mass of an individual BC particle is proportional to the area of the incandescence signal (McConnell et al., 2007).
The analysis of NH was performed by fluorescence within the CFA setup (Bigler et al., 2011). The melt water stream flowing at 1 mL min−1 was added to a reagent made from 1.29 g o-phthalaldehyde (C8H6O2), 60 mL ethanol, and 900 mL purified water (MilliQ) and to a buffer made from 35.8 g Na2HPO4•12H2O, 1000 mL MilliQ, 600 µL NaOH (>32 %), 100 µL HCHO (>37 %), and 0.8 g Na2SO3, following a 1 m mixing coil at 80 ∘C and a 0.2 m mixing coil at room temperature. The mixture was excited at 365 nm and detected at 400 nm by means of a photomultiplier-based detector (PMT-FL, FIAlab Instruments). Three standards were used for calibration based on a 100 mg L−1 ion chromatography multielement standard (VII, Certipur, Merck) and diluted to 24, 50, and 200 ppb NH. The melt rate of the CFA was kept between 4 and 5 cm min−1 with a response time of 12 s; the equivalent depth resolution of the NH dataset is less than 1 cm.
Based on the age scale released in Simonsen et al. (2019), the levoglucosan record covers the period from 0.089 to 5 kyr BP and a depth of 482 m, the BC record covers the period from 0.4 to 5 kyr BP and a depth of 482 m, and the NH covers the entire period back to 5 kyr BP.
2.2 Potential source regions of RECAP fluxes
To identify the potential forest fire regions able to influence the RECAP site, backward trajectories have been computed using the Hybrid Single-Particle Lagrangian Integrated Trajectory (HYSPLIT) model (Stein et al., 2015) with GDAS1 meteorological data. The GDAS1 dataset is available at 1×1∘ resolution. The HYSPLIT model was run using the PySPLIT Python package (Warner, 2018). Trajectories were calculated every hour in backward mode at three different altitudes (500, 1000, and 2000 m above Renland elevation: 2315 m a.s.l.) for the entire dataset available, that is, the period 2006–2019. A 7 d run time was chosen based on estimations of BC maximum residence time (Ramanathan and Carmichael, 2008; Cape et al., 2012).
The HYSPLIT modelling tool is useful for reconstructing the most likely transport areas for recent periods, and the information derived can be extended over a longer timescale (pre-industrial or Holocene), assuming the main atmospheric circulation mode has not changed significantly during those periods (Rubino et al., 2015). Stable isotope data (δ18O) suggest that the circulation pattern of air masses reaching the Greenland Ice Sheet has not significantly changed over the last 10 000 years (Vinther et al., 2006), which supports extending to the past back-trajectory analysis based on modern conditions.
2.3 Statistical approach
To investigate possible similarities with available time series, we estimated pairwise Pearson correlations among available records. Correlation coefficients were computed after interpolating all series to obtain a 20-year time resolution. Twenty-year bins were chosen in order to maximize time resolution among all time series limited by Northern Hemisphere temperature (Marcott et al., 2013). We investigated the correlation between our series with the NEEM levoglucosan flux (Zennaro et al., 2014); Northern Hemisphere temperatures as a tracer of the main climate variability (Marcott et al., 2013); RECAP δ18O (Hughes et al., 2020); and sedimentary charcoal influx composites as a regional fire reconstruction calculated over northern Europe, North America, and Siberia and more generally for the entire HLNH (high-latitude Northern Hemisphere, lat >55∘), available in the Global Charcoal Database (Blarquez et al., 2014).
To understand the mechanisms behind fire regime changes, we examine fire flux step changes and link them with climate and human history. To determine mean changes in time series, we conduct the offline change point analysis using the ruptures Python package (Truong et al., 2019). Three optimal breakpoints were found for levoglucosan flux using the PELT method; dynamic programming was then applied setting three breakpoints. As previously discussed, climate and especially summer temperatures have a central role in explaining the trends observed in RECAP fire reconstruction. However, other processes might be of relevance; in order to disentangle the contribution of climate in changing the fire regime, we calculate ratios between levoglucosan and BC fluxes with reconstructed Northern Hemisphere temperature from Marcott et al. (2013): a stable ratio suggests that climate is the main driver, while significant variations in the ratio suggest that additional processes are in place. We then perform change point analysis on ratios between normalized levoglucosan and BC fluxes and Northern Hemisphere temperature (Levo / T and BC / T).
3.1 Results from back-trajectory analysis
Seven-day-long back trajectories calculated with the HYSPLIT model over the period 2006–2019 suggest that the higher frequency of trajectories comes from the nearby areas with respect to the Renland site (Fig. 1), as also evidenced by Simonsen et al. (2019) and Maffezzoli et al. (2019) studying insoluble dust and sea ice, respectively. In addition to the area surrounding the Renland ice cap, the coastal area immediately to the north of the Renland site appears to be the most probable source region. The south-east and south-west coastal areas of Greenland and Iceland are also ice-free source areas which provide the densest and shortest trajectories considering the density of gridded points and travelling time. We define the region ranging 60–0∘ W in longitude and 90–55∘ N in latitude as the region with the most likely source areas of materials arriving at Renland (black box in Fig. 1), and we hereafter call it the high North Atlantic region (HNAR).
Other contributors of air masses are North America, northern Europe, and Siberia. Boreal forests of North America and Siberia are also possible sources of impurities, as well as northern Europe (Schüpbach et al., 2018); however they require a longer travel path for fire emissions to reach the Renland site compared to coastal Greenland and Iceland and are thus expected to carry only a minor contribution.
3.2 Levoglucosan, BC, and NH results
RECAP levoglucosan, BC, and NH profiles display distinct variability on centennial timescales and do not exhibit a clear trend over the past 5 kyr (Fig. S1). The levoglucosan concentrations vary from 0.006 to 0.1 ng g−1 with a mean (considering the whole 5 kyr record) of 0.033 ± 0.002 ng g−1, the BC concentration profile varies from 0.4 to 1.7 ng g−1 with a mean of 0.942 ± 0.006 ng g−1, and NH varies from 0.09 to 17 ng g−1 with a mean of 5.0 ± 0.2 ng g−1.
Since Levoglucosan, BC, and NH can be deposited in snow and glaciers by both wet and dry deposition (Stohl et al., 2007), fluxes were calculated as the product of concentration with annual accumulation and expressed in micrograms per square metre per year (µg m−2 yr−1). Renland accumulation (Hughes et al., 2020; Corella et al., 2019; Simonsen et al., 2019) shows a stable profile, indicating that wet deposition did not undergo drastic changes over the period covered by our record (Vinther et al., 2009). The levoglucosan flux (Fig. 3a) exhibits the same major features observed in the concentration record, indicating that variations in the levoglucosan series reflect changes in the atmospheric concentration, rather than a change in snow accumulation (Grieman et al., 2018). The levoglucosan flux shows high levels from 5 to 4.5 kyr BP, with 31.5 µg m−2 yr−1 on average. A rather stable deposition flux is determined between 4 and 2 kyr BP, while higher variability is determined during the period 2 to 1 kyr BP, with an average of 17 µg m−2 yr−1. Lower fluxes are found in the period 1 to 0.5 kyr BP, with an average of 10 µg m−2 yr−1. The BC flux is stable with an average of 464 µg m−2 yr−1 over the period 5 to 1 kyr BP (Fig. 3b). During this period some oscillations are detected with a higher depositional flux determined at ca. 3.6, 3, 2.5, and 2 kyr BP. A decrease in BC flux is determined, similarly to levoglucosan, after 1.1 kyr BP, with a value of 372 µg m−2 yr−1. The NH flux shows higher values from 5 to 4.6 kyr BP with an average of 2889 µg m−2 yr−1, a rather stable profile from 4 to 1.25 kyr BP (2387 µg m−2 yr−1 on average), and an abrupt decline at 2.7 kyr BP (Fig. 3c). Low values (2037 µg m−2 yr−1) are detected over the period 0.99–0.42 kyr BP with an abrupt increase during the last 200 years, with a maximum flux of 7915 µg m−2 yr−1.
3.3 Results from statistical analysis
Figure 2 shows the correlation matrix computed for the considered time series. The correlation between RECAP levoglucosan and BC fluxes is low but significant (r=0.21, p<0.01), as is that between BC and NH (r=0.27, p<0.01). The RECAP levoglucosan flux is significantly correlated with reconstructed Northern Hemisphere temperature (r=0.46, p<0.001) as well as with RECAP δ18O (r=0.33, p<0.01); the RECAP BC flux is also significantly correlated with NH temperature (r=0.32, p<0.001) and with RECAP δ18O (r=0.29, p<0.01). No statistically significant correlation has been determined between RECAP biomass burning fluxes and the regional sedimentary charcoal influx composites (Fig. 2).
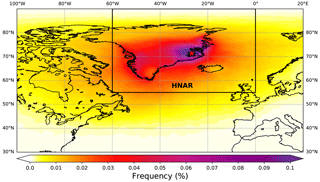
Figure 1Seven-day back trajectories of years 2006–2019 at 500, 1000, and 2000 m above Renland elevation, indicated as number of endpoints divided by number of trajectories. When more than one point of the same trajectory fall in the same grid cell, they are counted as one. The black box indicates the high North Atlantic region (HNAR), extending between 60–0∘ W in longitude and 90–55∘ N in latitude. The red star indicates the Renland site.
Breakpoints resulting from change point analysis are shown in Fig. 4 by a change of colour. As for the levoglucosan flux, two main step changes are found at 4.45 and 1.15 kyr, where the profile significantly decreases, while at 0.2 kyr BP it increases; regarding the BC flux, one strong step decline is found at 1.03 kyr BP, while for the NH flux the main step changes are found at 4.57, 4.37, and 0.12 kyr BP (Fig. 4a, c, e). It was not possible to detect the concomitant shift at 1.15 kyr BP in the NH flux due to an absence of data. We also perform change point analysis on ratios between normalized levoglucosan and BC fluxes and Northern Hemisphere temperature (Levo / T and BC / T in Fig. 4b, d). The ratio Levo / T presents step changes at 4.49, 1.19, and 0.37 kyr BP, while BC / T presents them at 4.41, 1.11, and 0.71 kyr BP. The mean shifts at around 1.1 kyr BP of Levo / T and BC / T differ by 100 years; however, while Levo / T strongly decreases at 4.5 kyr BP, BC / T increases.
4.1 Climate influence on fire regimes in the high North Atlantic region
As opposed to other Greenlandic ice cores such as NEEM, the RECAP core is retrieved at a coastal site; thus it is speculated to be more influenced by local sources in the Holocene (Simonsen et al., 2019). From back-trajectory analysis (Fig. 1) and the available literature (Corella et al., 2019; Simonsen et al., 2019) we infer that impurities arriving at the Renland site mostly originate in the HNAR, as defined in Sect. 2.2. Our record is therefore a useful archive for evaluating the fire history at the high latitudes of the North Atlantic.
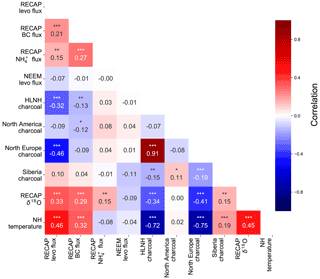
Figure 2Correlation matrix of 5 kyr records with resolution of 20 years. *** indicates p<0.01, ** indicates p<0.05, and * indicates p<0.1. The colour bar represents the correlation from −1 to 1.
Several studies suggest that climate is the main driver of global biomass burning (Marlon et al., 2008). Elevated summer temperatures and sustained droughts can affect fuel flammability and lead to increased global fire activity over seasonal to centennial timescales (Daniau et al., 2012). Climate conditions have also determined forest expansion (and glacier retreat), with positive effects on fuel moisture and a dampening effect on biomass burning (Feurdean et al., 2020b). Significant correlations of RECAP fire proxies with NH temperature during the past 5 kyr period (Fig. 2) suggest that climatic conditions play an important role in controlling forest fire activity in the HNAR. Fire has reduced throughout the late Holocene in parallel with progressively decreasing summer insolation in the Northern Hemisphere through the late Holocene (Power et al., 2008). Greater-than-present summer insolation resulted in warmer and drier summers in the Northern Hemisphere with increasing fire activity as shown by records from North America and Europe. Renland, being located at a high latitude, receives minimal or no insolation throughout the winter, meaning that summer insolation dominates (Hughes et al., 2020). Thus, summer solar input and temperature strongly influence fire activity in the HNAR.
High levels of levoglucosan and NH fluxes from 5 to 4.5 kyr BP could be linked to the warm NH temperatures of the mid-Holocene. In fact, Marcott et al. (2013) find warmer temperatures from 9.5 to 5.5 kyr B.P, followed by a cooling trend from 5.5 kyr BP onwards. Such a cooling trend could explain levoglucosan and NH decrease from 5 to 4.5 kyr BP. An additional source of NH fluxes, however, could also be soil emission from ice-free areas of Greenland, especially during summer when the seasonal ice melts (Fischer et al., 2015). The RECAP levoglucosan flux is high at 1.5 kyr BP, in concomitance with elevated RECAP summer δ18O (Hughes et al., 2020), and successively declines until 1 kyr BP, where values remain low until 200 years BP (Fig. 3). This agrees with the higher RECAP summer δ18O trend (Hughes et al., 2020). Marcott et al. (2013) and Mann et al. (2008) document a cooling trend from a warm interval (∼ 1.5–1 kyr BP) to a cool interval (∼ 0.5–0.1 kyr BP). The increase in levoglucosan flux over the last 200 years is most likely connected with the increase in NH temperatures and greenhouse gases. Charcoal records from the NH show a striking decrease in biomass burning from the late 19th century to mid-to-late 20th century (Marlon et al., 2008); however this is below the resolution of the RECAP fire tracer fluxes.
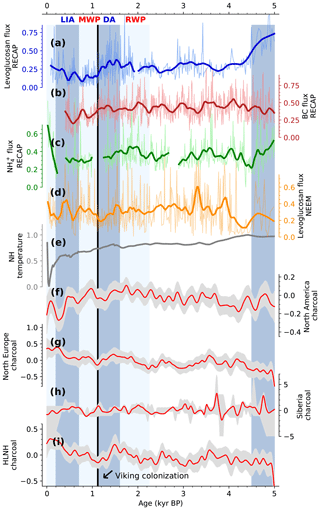
Figure 3Normalized 5 kyr time series of (a) RECAP levoglucosan flux; (b) RECAP BC flux; (c) RECAP NH flux; (d) NEEM levoglucosan flux (Zennaro et al., 2015); (e) NH temperature reconstruction (90–30∘ latitude) (Marcott et al., 2013); and (f, g, h, i) charcoal composite record of North America, northern Europe, Siberia, and the Northern Hemisphere (lat > 55∘), respectively (red lines), with 0.05 and 0.95 confidence levels (grey areas). The black line indicates the time of the Viking colonization of Iceland. Age scale is indicated as 1000 years before 2000.
RECAP levoglucosan and BC fluxes do not correlate with NEEM levoglucosan flux (Fig. 2), suggesting a different influence area. While Zennaro et al. (2014) suggest that NEEM fire sources are mainly North American and Eurasian boreal sources, RECAP is a coastal core, and local sources like coastal Greenland and Iceland likely have a major influence. Furthermore, the 15 kyr NEEM levoglucosan record shows a maximum in biomass burning at ∼ 3.5 to 1.5 kyr BP, associated with high fire levels in North America and Europe and with wetter conditions in North America (Zennaro et al., 2015). Such a trend is not found for RECAP proxies. For the last 2 kyr, a declining trend starting at ∼ 1.5 kyr BP is shared by NEEM and RECAP fire proxies; however, during the Medieval Climate Anomaly (1.2–0.8 kyr BP) NEEM levoglucosan increases again, while RECAP fire proxies stay low. The weak similarity between NEEM and RECAP fire proxies further suggests that the two sites have different source areas.
Based on the Global Charcoal Database, fire regime reconstructions in the Northern Hemisphere have been compiled for the North America, northern Europe, and Siberia regions, as well as for the whole high-latitude Northern Hemisphere (lat >55∘), and some of these could contribute to the RECAP levoglucosan flux. Our statistical analysis suggests weak correlations between RECAP biomass burning fluxes and charcoal composites from North America (Fig. 2). RECAP levoglucosan and BC are significantly but negatively correlated with HLNH and northern Europe composite charcoal records. The negative correlation does not explain the trend observed in the RECAP records but suggests instead that different fire regimes might exist between northern Europe and the HNAR. General circulation patterns and storm tracks, which mostly move from west to east, likely move European fire proxies away from Renland, further supporting the hypothesis that northern Europe fire history is not captured by RECAP ice.
Two regions that might influence fire proxy deposition at the Renland site remain to be considered: Siberia and Iceland. Past information on fire activity in the Siberia region comes from few charcoal datasets and ice core records. From the increasing abundance of charcoal morphologies of all types in Plotnikovo Mire, frequent local fires are found between 4.8 and 3.9 cal yr BP and 2.8 and 1.5 kyr BP (Feurdean et al., 2020a), while Eichler et al. (2011) find increased fire activity between 400 and 320 BP from ice core nitrate and potassium and charcoal. Similar to the regions of North America and northern Europe, no correlation is found between RECAP ice core fire records and the composite charcoal record for the Siberia region. For Iceland, however, no fire history record is available to our knowledge. The contribution from Greenland can be only considered in warm periods of the Holocene when the ice sheet retreats and vegetation of grass and shrublands grows along the coasts.
We suggest that the main source of fire markers that are deposited at Renland, together with eastern Greenland, is Iceland. Our hypothesis is based on the absence of a correlation between fire tracer fluxes and any charcoal record from the regions investigated as possible sources, the low correlation with the NEEM levoglucosan flux, and the results from back-trajectory analysis. Iceland has high aeolian activity driven by climate, volcanic activity, and glacial sediment supply. Atmospheric low-pressure systems are common, sometimes referred to as the “Icelandic low”, which frequently result in relatively high wind speeds (Arnalds et al., 2016). It is thus likely that RECAP fire proxies reflect fire emissions from Iceland rather than a hemispheric trend. The fire history of Iceland has never been documented, and the RECAP ice core might represent the first record preserving past Icelandic fire changes. The significant link between levoglucosan and BC with temperature reconstruction as well as RECAP δ18O suggests that the change of the Icelandic fire regime was mostly driven by climate fluctuations. Also, although the Icelandic territory is characterized by high humidity and frequent precipitation (Ólafsson et al., 2007), which could limit fire propagation, natural fires might be triggered more easily there than in other regions due to the large number of volcanoes and associated eruptions (Butwin et al., 2018).
4.2 Additional processes explaining fire proxy variability
Global climate alone may not be the only driver of fire activity. Additional processes are suggested to have an impact on the fire regime (Marlon et al., 2008), such as anthropogenic activities including land clearance; ice sheet advance/retreat; and peat fires accentuated by permafrost thawing, altering the temporal and spatial structure of fuel and the frequency of ignitions since the early Holocene (Pfeiffer et al., 2013; Vannière et al., 2016; Andela et al., 2017).
From change point analysis conducted on fire fluxes and on ratios with temperature we identify three main changes at approximately 4.5, 1.1, and 0.2 kyr BP, found to be common to more than one time series. In the following sections we formulate hypotheses about possible processes inducing fire regime variability.
4.2.1 The period 5–4.5 kyr BP: decline in summer insolation
One possible process explaining decreasing levoglucosan and NH fluxes during the period 5–4.5 kyr BP is the monotonic Holocene decline in Northern Hemisphere summer insolation. Cooler conditions caused cryosphere expansion and progressively a lowered equilibrium-line altitude in the Iceland highlands, as well as in eastern Greenland, the Baffin Islands, western Svalbard, and western Norway (Geirsdóttir et al., 2019). High-resolution lacustrine records (Geirsdóttir et al., 2013) indicate that, despite the monotonic decline in summer insolation, Iceland’s landscape changes and ice cap expansions were nonlinear with abrupt changes occurring at 5, 4.5–4.0, 3, and 1.5 kyr BP. It is possible that the abrupt decline in levoglucosan and NH fluxes at 4.5 kyr BP was the result of consistent glacier advance and consequent reduction of vegetation.
Biomass burning is linked not only to climate but also to vegetation composition, with denser vegetation providing greater fuel availability and hence high fire intensity. Evidence of dense vegetation during the mid-Holocene, associated with a warm climate, is reported both in eastern Greenland and Iceland. Pollen records show the presence of dense dwarf shrubs of low Arctic Betula nana in the Scoresby Sund area (Funder, 1978) and Basaltsø (Wagner et al., 2000) from 8 to 5 kyr BP, indicating warm and dry conditions in eastern Greenland. As regards Iceland, Eddudóttir (2016) reports birch woodland expansion from 7.9 kyr BP from high Betula pubescens pollen counts in a lake record from the north-west highlands. Woodlands then start to decline from 6 kyr BP (Hallsdóttir and Caseldine, 2005). Cooler summers after 5 kyr BP, leading to sparse vegetation cover, may thus be the cause of the reduced fire regime in the HNAR.
RECAP levoglucosan and BC fluxes have different trends in the period 5–4.5 kyr BP (Figs. 3, 4). One possible explanation is that during the early Holocene (8–5 kyr BP) larger areas of the Greenland coasts and Iceland were dry (Wagner et al., 2000) and ice-free (Geirsdóttir et al., 2009) and thus subject to wildfires linked to smoldering of peats. Such wildfires are fuelled by the organic matter contained in the soil and are accentuated by the melting of permafrost. Levoglucosan, being emitted by low-temperature fires, might also capture burning grasslands and shrubs (Kehrwald et al., 2020) that grow densely in the HNAR during the early Holocene. Peat and grass fires along the Greenland coasts could explain the higher fluxes of levoglucosan and ammonium in this period. BC, in contrast, is mostly emitted under flaming conditions (Legrand et al., 2016), and there is uncertainty regarding the quality of the BC proxy in tracing peat fires (Jayarathne et al., 2018). Further studies, however, are necessary to understand the mechanisms explaining the diverging behaviour of levoglucosan and BC.
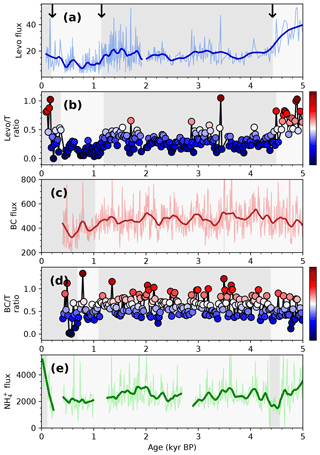
Figure 4Change point analysis of (a) levoglucosan flux, (b) ratio of normalized levoglucosan and NH temperature, (c) BC flux, (d) ratio of normalized levoglucosan and NH temperature, and (e) NH flux. The change of background colour indicates the mean shift. The significant breakpoints are indicated by a change of colour. The changes in common for more than one time series are indicated by arrows.
The latter part of the Holocene is characterized by unstable conditions in the HNAR. Eastern Greenland vegetation becomes progressively sparser after 5 kyr BP, with a rise of Salix- and Cassiope-dominated poor dwarf shrub heaths, indicating moist and cool conditions (Wagner et al., 2000). Environmental instability starting from 4.2 kyr BP is reported in north-west Iceland, indicated by a sparse presence of Betula pubescens (Eddudóttir, 2016). Evidence of Sorbus cf. aucuparia pollen suggests that shade-intolerant herbs were replacing birch woodlands (Hallsdóttir and Caseldine, 2005), resulting in sparser, more open vegetation. Stable RECAP fire fluxes from 4.5 kyr BP onwards may thus reflect cooler climate conditions and sparser vegetation in the HNAR. Woodland regeneration in Iceland becomes evident at 1.6 kyr BP (Hallsdóttir and Caseldine, 2005) and likely reflects the coincidently increased RECAP levoglucosan flux.
4.2.2 Change at 1.1 kyr BP: Viking colonization of Iceland
The change of levoglucosan and BC fluxes, Levo / T, and BC / T at 1.1 kyr BP may be explained by human activities in Iceland (Fig. 4). Iceland was colonized from Scandinavia and Britain in the late 9th century CE (Vésteinsson and McGovern, 2012) and thereafter underwent a rapid and comprehensive deforestation in a matter of decades, during which large areas completely lost their woodland cover after the arrival of the first settlers (Erlendsson and Edwards, 2009; Streeter et al., 2015). The early settlers cleared land mainly through tree felling, as inferred from the absence of charcoal layers, which would indicate the use of fire through either forest clearance or application of slash-and-burn techniques (Trbojević, 2016). Grass heaths and mires expanded at the expense of the woodlands, as inferred from pollen counts (Hallsdóttir and Caseldine, 2005).
The land clearance, together with livestock overgrazing, resulted in the loss of more than 25 % of the vegetation cover and in soil erosion (Haraldsson and Ólafsdóttir, 2003; Erlendsson and Edwards, 2009; Streeter et al., 2015), leading to the reduction of the areas which could support vegetation and that would be burnt in the event of a fire being triggered. We thus suggest that the decline of RECAP fire proxies at 1.1 kyr BP is a consequence of the reduction of fuel availability in Iceland. Although the climatic cooling, strengthened from the year 700 BP onwards, can to a great extent explain the observed reduction in forest fires, the human contribution must also be accounted for. It is likely that the inappropriate human management of natural resources acted in parallel to climatic cooling, causing what is recognized to be one of the first environmental disasters.
4.2.3 The last 200 years: global increase in temperatures and land conversion rates
The levoglucosan flux and Levo / T shift over the last few centuries (Fig. 4a, b). The levoglucosan flux changes 200 years BP, while Levo / T changes earlier at 370 BP. The shift of levoglucosan to higher values is probably related to increasing global temperatures and is associated with increased dwarf shrub pollen percentages at the sediment surface in eastern Greenland (Wagner et al., 2000). The last decades of the 19th century were the period of maximum population expansion and land cover conversion for agricultural purposes in many areas of the Northern Hemisphere (Marlon et al., 2008). Zennaro et al. (2014) found relatively high levoglucosan values in the NEEM ice core during the last 2 centuries, although a clear assessment was not possible due to lack of data. Several charcoal records from North America, Siberia, and Europe evidence an increase since ∼ 200 BP (as also reported in Fig. 4f, g, i), which has been attributed both to climate and to increasing use of fire for land clearance (Marlon et al., 2008, 2016).
The abrupt increase of NH over the last 120 years may be attributed to anthropogenic emissions. Wendl et al. (2015) argued that trends in NH concentrations in the Lomonosovfonna-09 ice core (Svalbard) after 60 BP indicate a strong anthropogenic influence mainly from NH3 emissions from agriculture and livestock in Eurasia. No BC data are available for the last 500 years; however, high values of BC flux are expected since several shallow cores from Svalbard and Greenland show broad concentration maxima starting from 140 BP and are attributed to anthropogenic emissions (Osmont et al., 2018).
This paper provides 5 kyr records of the fire proxies levoglucosan, BC, and NH in the RECAP ice core in Greenland.
From back-trajectory analysis and the comparison with regional fire reconstructions based on charcoal records, we find that the most likely source area of impurities arriving at Renland is the high North Atlantic region (ranging from the longitudes 60–0∘ W and the latitudes 90–55∘ N) and comprehends the Greenland Ice Sheet, the coasts of Greenland, and Iceland. Iceland, in particular, might have had a great influence in driving fire regime changes in RECAP fire proxies due to its position with respect to the Renland site and to the presence of large forested areas throughout the Holocene. In the Northern Hemisphere, Iceland is also the only region which is not up until now covered by fire reconstructions, and RECAP fire proxies could enable the first reconstruction of the past Icelandic fire regime.
We find that climate variability is the main control of changes in the High North Atlantic fire regime, especially regarding temperature driven by summer insolation. A downturn of levoglucosan and NH fluxes at 4.5 kyr BP may be associated with the monotonic Holocene decline in Northern Hemisphere summer insolation that resulted in cooler conditions, cryosphere expansion, and vegetation reduction in the HNAR. BC shows a different trend in this period, possibly explained by wildfires linked to smoldering of peats along the Greenland coasts due to warmer temperatures and melting of permafrost. While levoglucosan and NH can record peat fires, BC is not a good proxy because it is emitted during flaming conditions when biomass burns. Further studies are necessary to confirm our hypothesis. During the last millennium the fire regime may have been influenced by the human impact on the Icelandic environment, which was uninhabited before the arrival of the Vikings. We hypothesize that the massive land clearing and the active fire suppression probably led to a reduction of wildfires. The human impact on the fire regime probably became more prominent over the last centuries due to population expansion and increases in land cover conversion rates for agricultural purposes.
The dataset will be available in the Pangaea database.
The supplement related to this article is available online at: https://doi.org/10.5194/cp-17-1533-2021-supplement.
DS and AS designed the research. MDCVH, RZ, and EB performed the levoglucosan analysis. HAK, MS, BV, and PV processed the ice core samples, ran the ammonium analysis, and helped in the data interpretation. RE performed black carbon analysis. DS and NM performed and ran the back-trajectory analysis. DB, CB, CT, OV, AS, and DS contributed to the data interpretation. DS and AS wrote the manuscript with input from all the authors.
The authors declare that they have no conflict of interest.
Publisher's note: Copernicus Publications remains neutral with regard to jurisdictional claims in published maps and institutional affiliations.
The RECAP ice coring effort was financed by the Danish Research Council through a Sapere Aude grant, the NSF through the Division of Polar Programs, the Alfred Wegener Institute, and the European Research Council (ERC) under the European Community’s Seventh Framework Programme (FP7/2007-2013)/ERC grant agreement 610055 through the Ice2Ice project. This project has received funding from the European Union's Horizon 2020 research and innovation programme under grant agreement no. 689443 via project iCUPE (Integrative and Comprehensive Understanding on Polar Environments). The research leading to these results has received funding from the European Union’s Seventh Framework Programme (specific programme: “Ideas”; ERC Advanced Grant) under grant agreement no. 267696 “EARLYhumanIMPACT”. We acknowledge the help of ELGA LabWater, which provides the ultrapure water used for levoglucosan analysis at Ca' Foscari University of Venice. We thank the two anonymous reviewers, whose comments/suggestions helped improve and clarify this paper.
This research has been supported by the FP7 Ideas: European Research Council (grant nos. 610055 and 267696) and the iCUPE (Integrative and Comprehensive Understanding on Polar Environments) – European Union's Horizon 2020 (grant no. 689443).
This paper was edited by Anne-Laure Daniau and reviewed by Angelica Feurdean and one anonymous referee.
Andela, N., Morton, D. C., Giglio, L., Chen, Y., van der Werf, G. R., Kasibhatla, P. S., DeFries, R. S., Collatz, G. J., Hantson, S., Kloster, S., Bachelet, D., Forrest, M., Lasslop, G., Li, F., Mangeon, S., Melton, J. R., Yue, C., and Randerson, J. T.: A human-driven decline in global burned area, Science, 356, 1356–1362, https://doi.org/10.1126/science.aal4108, 2017. a, b
Arnalds, O., Dagsson-Waldhauserova, P., and Olafsson, H.: The Icelandic volcanic aeolian environment: Processes and impacts – A review, Aeolian Research, 20, 176–195, https://doi.org/10.1016/j.aeolia.2016.01.004, 2016. a
Barrett, T., Ponette-González, A., Rindy, J., and Weathers, K.: Wet deposition of black carbon: A synthesis, Atmos. Environ., 213, 558–567, https://doi.org/10.1016/j.atmosenv.2019.06.033, 2019. a
Battistel, D., Kehrwald, N. M., Zennaro, P., Pellegrino, G., Barbaro, E., Zangrando, R., Pedeli, X. X., Varin, C., Spolaor, A., Vallelonga, P. T., Gambaro, A., and Barbante, C.: High-latitude Southern Hemisphere fire history during the mid- to late Holocene (6000–750 BP), Clim. Past, 14, 871–886, https://doi.org/10.5194/cp-14-871-2018, 2018. a, b
Bhattarai, H., Saikawa, E., Wan, X., Zhu, H., Ram, K., Gao, S., Kang, S., Zhang, Q., Zhang, Y., Wu, G., Wang, X., Kawamura, K., Fu, P., and Cong, Z.: Levoglucosan as a tracer of biomass burning: Recent progress and perspectives, Atmos. Res., 220, 20–33, https://doi.org/10.1016/j.atmosres.2019.01.004, 2019. a
Bigler, M., Svensson, A., Kettner, E., Vallelonga, P., Nielsen, M., and Steffensen, J.: Optimization of High-Resolution Continuous Flow Analysis for Transient Climate Signals in Ice Cores, Environ. Sci. Technol., 45, 4483–4489, https://doi.org/10.1021/es200118j, 2011. a, b
Blarquez, O., Vannière, B., Marlon, J. R., Daniau, A.-L., Power, M. J.,
Brewer, S., and Bartlein, P. J.: paleofire
: An R package to
analyse sedimentary charcoal records from the Global Charcoal Database
to reconstruct past biomass burning, Comput. Geosci., 72, 255–261, https://doi.org/10.1016/j.cageo.2014.07.020, 2014. a, b
Bond, T., Doherty, S., Fahey, D., Forster, P., Berntsen, T., DeAngelo, B., Flanner, M., Ghan, S., Kärcher, B., Koch, D., Kinne, S., Kondo, Y., Quinn, P., Sarofim, M., Schultz, M., Michael, S., Venkataraman, C., Zhang, H., Zhang, S., and Zender, C.: Bounding the role of black carbon in the climate system: A Scientific assessment, J. Geophys. Res.-Atmos., 118, 5380–5552, https://doi.org/10.1002/jgrd.50171, 2013. a
Bowman, D. M. J. S., Balch, J. K., Artaxo, P., Bond, W. J., Carlson, J. M., Cochrane, M. A., D'Antonio, C. M., DeFries, R. S., Doyle, J. C., Harrison, S. P., Johnston, F. H., Keeley, J. E., Krawchuk, M. A., Kull, C. A., Marston, J. B., Moritz, M. A., Prentice, I. C., Roos, C. I., Scott, A. C., Swetnam, T. W., van der Werf, G. R., and Pyne, S. J.: Fire in the Earth System, Science, 324, 481–484, https://doi.org/10.1126/science.1163886, 2009. a, b
Butwin, M., Löwis, S., Pfeffer, M., and Thorsteinsson, T.: The Effects of Volcanic Eruptions on the Frequency of Particulate Matter Suspension Events in Iceland, J. Aerosol Sci., 128, 99–113, https://doi.org/10.1016/j.jaerosci.2018.12.004, 2018. a
Cape, J., Coyle, M., and Dumitrean, P.: The atmospheric lifetime of black carbon, Atmos. Environ., 59, 256–263, https://doi.org/10.1016/j.atmosenv.2012.05.030, 2012. a, b
Chuvieco, E., Mouillot, F., van der Werf, G. R., San Miguel, J., Tanase, M., Koutsias, N., García, M., Yebra, M., Padilla, M., Gitas, I., Heil, A., Hawbaker, T. J., and Giglio, L.: Historical background and current developments for mapping burned area from satellite Earth observation, Remote Sens. Environ., 225, 45–64, https://doi.org/10.1016/j.rse.2019.02.013, 2019. a
Corella, J. P., Maffezzoli, N., Cuevas, C. A., Vallelonga, P., Spolaor, A., Cozzi, G., Müller, J., Vinther, B., Barbante, C., Kjær, H. A., Edwards, R., and Saiz-Lopez, A.: Holocene atmospheric iodine evolution over the North Atlantic, Clim. Past, 15, 2019–2030, https://doi.org/10.5194/cp-15-2019-2019, 2019. a, b
Daniau, A., Bartlein, P. J., Harrison, S. P., Prentice, I. C., Brewer, S., Friedlingstein, P., Harrison-Prentice, T. I., Inoue, J., Izumi, K., Marlon, J. R., Mooney, S., Power, M. J., Stevenson, J., Tinner, W., Andrič, M., Atanassova, J., Behling, H., Black, M., Blarquez, O., Brown, K. J., Carcaillet, C., Colhoun, E. A., Colombaroli, D., Davis, B. A. S., D'Costa, D., Dodson, J., Dupont, L., Eshetu, Z., Gavin, D. G., Genries, A., Haberle, S., Hallett, D. J., Hope, G., Horn, S. P., Kassa, T. G., Katamura, F., Kennedy, L. M., Kershaw, P., Krivonogov, S., Long, C., Magri, D., Marinova, E., McKenzie, G. M., Moreno, P. I., Moss, P., Neumann, F. H., Norström, E., Paitre, C., Rius, D., Roberts, N., Robinson, G. S., Sasaki, N., Scott, L., Takahara, H., Terwilliger, V., Thevenon, F., Turner, R., Valsecchi, V. G., Vannière, B., Walsh, M., Williams, N., and Zhang, Y.: Predictability of biomass burning in response to climate changes, Global Biogeochem. Cy., 26, GB4007, https://doi.org/10.1029/2011GB004249, 2012. a
Doughty, C. E.: Preindustrial Human Impacts on Global and Regional Environment, Annu. Rev. Environ. Resour., 38, 503–527, https://doi.org/10.1146/annurev-environ-032012-095147, 2013. a
Eddudóttir, S.: Holocene Environmental Change in Northwest Iceland, Ph.D. thesis, University of Iceland, 2016. a, b
Eichler, A., Tinner, W., Brütsch, S., Olivier, S., Papina, T., and Schwikowski, M.: An ice-core based history of Siberian forest fires since AD 1250, Quaternary Sci. Rev., 30, 1027–1034, https://doi.org/10.1016/j.quascirev.2011.02.007, 2011. a
Erlendsson, E. and Edwards, K. J.: The timing and causes of the final pre-settlement expansion of Betula pubescens in Iceland, The Holocene, 19, 1083–1091, https://doi.org/10.1177/0959683609341001, 2009. a, b
Feurdean, A., Florescu, G., Tanţău, I., Vannière, B., Diaconu, A.-C., Pfeiffer, M., Warren, D., Hutchinson, S. M., Gorina, N., Gałka, M., and Kirpotin, S.: Recent fire regime in the southern boreal forests of western Siberia is unprecedented in the last five millennia, Quaternary Sci. Rev., 244, 106495, https://doi.org/10.1016/j.quascirev.2020.106495, 2020a. a, b
Feurdean, A., Vannière, B., Finsinger, W., Warren, D., Connor, S. C., Forrest, M., Liakka, J., Panait, A., Werner, C., Andrič, M., Bobek, P., Carter, V. A., Davis, B., Diaconu, A.-C., Dietze, E., Feeser, I., Florescu, G., Gałka, M., Giesecke, T., Jahns, S., Jamrichová, E., Kajukało, K., Kaplan, J., Karpińska-Kołaczek, M., Kołaczek, P., Kuneš, P., Kupriyanov, D., Lamentowicz, M., Lemmen, C., Magyari, E. K., Marcisz, K., Marinova, E., Niamir, A., Novenko, E., Obremska, M., Pędziszewska, A., Pfeiffer, M., Poska, A., Rösch, M., Słowiński, M., Stančikaitė, M., Szal, M., Święta-Musznicka, J., Tanţău, I., Theuerkauf, M., Tonkov, S., Valkó, O., Vassiljev, J., Veski, S., Vincze, I., Wacnik, A., Wiethold, J., and Hickler, T.: Fire hazard modulation by long-term dynamics in land cover and dominant forest type in eastern and central Europe, Biogeosciences, 17, 1213–1230, https://doi.org/10.5194/bg-17-1213-2020, 2020b. a
Fischer, H., Schüpbach, S., Gfeller, G., Bigler, M., Röthlisberger, R., Erhardt, T., Stocker, T. F., Mulvaney, R., and Wolff, E. W.: Millennial changes in North American wildfire and soil activity over the last glacial cycle, Nat. Geosci., 8, 723–727, https://doi.org/10.1038/ngeo2495, 2015. a, b
Funder, S.: Holocene stratigraphy and vegetation history in the Scoresby Sund area, East Greenland, Bulletin – Groenlands Geologiske Undersoegelse, 129, 1–76, available at: https://inis.iaea.org/search/search.aspx?orig_q=RN:10462964 (last access: 14 July 2021), 1978. a
Gambaro, A., Zangrando, R., Gabrielli, P., Barbante, C., and Cescon, P.: Direct Determination of Levoglucosan at the Picogram per Milliliter Level in Antarctic Ice by High-Performance Liquid Chromatography/Electrospray Ionization Triple Quadrupole Mass Spectrometry, Anal. Chem., 80, 1649–1655, https://doi.org/10.1021/ac701655x, 2008. a, b, c
Geirsdóttir, A., Miller, G. H., Axford, Y., and Sædís Ólafsdóttir: Holocene and latest Pleistocene climate and glacier fluctuations in Iceland, Quaternary Sci. Rev., 28, 2107–2118, https://doi.org/10.1016/j.quascirev.2009.03.013, 2009. a
Geirsdóttir, A., Miller, G., Larsen, D., and Olafsdottir, S.: Abrupt Holocene climate transitions in the northern North Atlantic region recorded by synchronized lacustrine records in Iceland, Quaternary Sci. Rev., 70, 48–62, https://doi.org/10.1016/j.quascirev.2013.03.010, 2013. a
Geirsdóttir, Á., Miller, G. H., Andrews, J. T., Harning, D. J., Anderson, L. S., Florian, C., Larsen, D. J., and Thordarson, T.: The onset of neoglaciation in Iceland and the 4.2 ka event, Clim. Past, 15, 25–40, https://doi.org/10.5194/cp-15-25-2019, 2019. a
Grieman, M. M., Aydin, M., Isaksson, E., Schwikowski, M., and Saltzman, E. S.: Aromatic acids in an Arctic ice core from Svalbard: a proxy record of biomass burning, Clim. Past, 14, 637–651, https://doi.org/10.5194/cp-14-637-2018, 2018. a, b
Hallsdóttir, M. and Caseldine, C. J.: 14. The Holocene vegetation history of Iceland, state-of-the-art and future research, in: Iceland – Modern Processes and Past Environments, vol. 5 of Developments in Quaternary Sciences, pp. 319–334, Elsevier, https://doi.org/10.1016/S1571-0866(05)80016-8, 2005. a, b, c, d
Haraldsson, H. and Ólafsdóttir, R.: Simulating vegetation cover dynamics with regards to long-term climatic variations in sub-Arctic landscapes, Global Planet. Change, 38, 313–325, https://doi.org/10.1016/S0921-8181(03)00114-0, 2003. a
Hoffmann, D., Tilgner, A., Iinuma, Y., and Herrmann, H.: Atmospheric stability of levoglucosan: a detailed laboratory and modeling study, Environ. Sci. Technol., 44, 694–699, https://doi.org/10.1021/es902476f, 2010. a
Hughes, A. G., Jones, T. R., Vinther, B. M., Gkinis, V., Stevens, C. M., Morris, V., Vaughn, B. H., Holme, C., Markle, B. R., and White, J. W. C.: High-frequency climate variability in the Holocene from a coastal-dome ice core in east-central Greenland, Clim. Past, 16, 1369–1386, https://doi.org/10.5194/cp-16-1369-2020, 2020. a, b, c, d, e
Jayarathne, T., Stockwell, C. E., Gilbert, A. A., Daugherty, K., Cochrane, M. A., Ryan, K. C., Putra, E. I., Saharjo, B. H., Nurhayati, A. D., Albar, I., Yokelson, R. J., and Stone, E. A.: Chemical characterization of fine particulate matter emitted by peat fires in Central Kalimantan, Indonesia, during the 2015 El Niño, Atmos. Chem. Phys., 18, 2585–2600, https://doi.org/10.5194/acp-18-2585-2018, 2018. a
Kehrwald, N. M., Jasmann, J. R., Dunham, M. E., Ferris, D. G., Osterberg, E. C., Kennedy, J., Havens, J., Barber, L. B., and Fortner, S. K.: Boreal blazes: biomass burning and vegetation types archived in the Juneau Icefield, Environ. Res. Lett., 15, 085005, https://doi.org/10.1088/1748-9326/ab8fd2, 2020. a
Keywood, M., Kanakidou, M., Stohl, A., Dentener, F., Grassi, G., Meyer, C. P., Torseth, K., Edwards, D., Thompson, A. M., Lohmann, U., and Burrows, J.: Fire in the Air: Biomass Burning Impacts in a Changing Climate, Crit. Rev. Environ. Sci. Technol., 43, 40–83, https://doi.org/10.1080/10643389.2011.604248, 2013. a
Legrand, M., McConnell, J., Fischer, H., Wolff, E. W., Preunkert, S., Arienzo, M., Chellman, N., Leuenberger, D., Maselli, O., Place, P., Sigl, M., Schüpbach, S., and Flannigan, M.: Boreal fire records in Northern Hemisphere ice cores: a review, Clim. Past, 12, 2033–2059, https://doi.org/10.5194/cp-12-2033-2016, 2016. a, b, c, d, e, f
Lim, S., Faïn, X., Ginot, P., Mikhalenko, V., Kutuzov, S., Paris, J.-D., Kozachek, A., and Laj, P.: Black carbon variability since preindustrial times in the eastern part of Europe reconstructed from Mt. Elbrus, Caucasus, ice cores, Atmos. Chem. Phys., 17, 3489–3505, https://doi.org/10.5194/acp-17-3489-2017, 2017. a
Maffezzoli, N., Vallelonga, P., Edwards, R., Saiz-Lopez, A., Turetta, C., Kjær, H. A., Barbante, C., Vinther, B., and Spolaor, A.: A 120 000-year record of sea ice in the North Atlantic?, Clim. Past, 15, 2031–2051, https://doi.org/10.5194/cp-15-2031-2019, 2019. a, b
Mann, M. E., Zhang, Z., Hughes, M. K., Bradley, R. S., Miller, S. K., Rutherford, S., and Ni, F.: Proxy-based reconstructions of hemispheric and global surface temperature variations over the past two millennia, P. Natl. Acad. Sci., 105, 13252–13257, https://doi.org/10.1073/pnas.0805721105, 2008. a
Marcott, S., Shakun, J., Clark, P., and Mix, A.: A Reconstruction of Regional and Global Temperature for the Past 11,300 Years, Science (New York, N.Y.), 339, 1198–1201, https://doi.org/10.1126/science.1228026, 2013. a, b, c, d, e, f
Marlon, J. R., Bartlein, P. J., Carcaillet, C., Gavin, D. G., Harrison, S. P., Higuera, P. E., Joos, F., Power, M. J., and Prentice, I. C.: Climate and human influences on global biomass burning over the past two millennia, Nat. Geosci., 1, 697–702, https://doi.org/10.1038/ngeo313, 2008. a, b, c, d, e, f, g, h, i
Marlon, J. R., Bartlein, P. J., Daniau, A.-L., Harrison, S. P., Maezumi, S. Y., Power, M. J., Tinner, W., and Vanniére, B.: Global biomass burning: a synthesis and review of Holocene paleofire records and their controls, Quaternary Sci. Rev., 65, 5–25, https://doi.org/10.1016/j.quascirev.2012.11.029, 2013. a, b, c
Marlon, J. R., Kelly, R., Daniau, A.-L., Vannière, B., Power, M. J., Bartlein, P., Higuera, P., Blarquez, O., Brewer, S., Brücher, T., Feurdean, A., Romera, G. G., Iglesias, V., Maezumi, S. Y., Magi, B., Courtney Mustaphi, C. J., and Zhihai, T.: Reconstructions of biomass burning from sediment-charcoal records to improve data–model comparisons, Biogeosciences, 13, 3225–3244, https://doi.org/10.5194/bg-13-3225-2016, 2016. a, b
McConnell, J. R., Edwards, R., Kok, G. L., Flanner, M. G., Zender, C. S., Saltzman, E. S., Banta, J. R., Pasteris, D. R., Carter, M. M., and Kahl, J. D. W.: 20th-Century Industrial Black Carbon Emissions Altered Arctic Climate Forcing, Science, 317, 1381–1384, https://doi.org/10.1126/science.1144856, 2007. a, b, c, d
Molinari, C., Lehsten, V., Blarquez, O., Carcaillet, C., Davis, B. A. S., Kaplan, J. O., Clear, J., and Bradshaw, R. H. W.: The climate, the fuel and the land use: Long-term regional variability of biomass burning in boreal forests, Glob. Change Biol., 24, 4929–4945, https://doi.org/10.1111/gcb.14380, 2018. a
Ólafsson, H., Furger, M., and Brümmer, B.: The weather and climate of Iceland, Meteorol. Z., 16, 5–8, https://doi.org/10.1127/0941-2948/2007/0185, 2007. a
Osmont, D., Wendl, I. A., Schmidely, L., Sigl, M., Vega, C. P., Isaksson, E., and Schwikowski, M.: An 800-year high-resolution black carbon ice core record from Lomonosovfonna, Svalbard, Atmos. Chem. Phys., 18, 12777–12795, https://doi.org/10.5194/acp-18-12777-2018, 2018. a
Osmont, D., Sigl, M., Eichler, A., Jenk, T. M., and Schwikowski, M.: A Holocene black carbon ice-core record of biomass burning in the Amazon Basin from Illimani, Bolivia, Clim. Past, 15, 579–592, https://doi.org/10.5194/cp-15-579-2019, 2019. a
Pfeiffer, M., Spessa, A., and Kaplan, J. O.: A model for global biomass burning in preindustrial time: LPJ-LMfire (v1.0), Geosci. Model Dev., 6, 643–685, https://doi.org/10.5194/gmd-6-643-2013, 2013. a
Power, M., Marlon, J., Ortiz, N., Bartlein, P., Harrison, S., Mayle, F., Ballouche, A., Bradshaw, R., Carcaillet, C., Cordova, C., Mooney, S., Moreno, P., Prentice, I., Thonicke, K., Tinner, W., Whitlock, C., Zhang, Y., Zhao, Y., Ali, A., Anderson, R., Beer, R., Behling, H., Briles, C., Brown, K., Brunelle, A., Bush, M., Camill, P., Chu, G., Clark, J., Colombaroli, D., Connor, S., Daniau, A., Daniels, M., Dodson, J., Doughty, E., Edwards, M., Finsinger, W., Foster, D., Frechette, J., Gaillard, M., Gavin, D., Gobet, E., Haberle, S., Hallett, D., Higuera, P., Hope, G., Horn, S., Inoue, J., Kaltenrieder, P., Kennedy, L., Kong, Z., Larsen, C., Long, C., Lynch, J., Lynch, E., McGlone, M., Meeks, S., Mensing, S., Meyer, G., Minckley, T., Mohr, J., Nelson, D., New, J., Newnham, R., Noti, R., Oswald, W., Pierce, J., Richard, P., Rowe, C., Sanchez Goñi, M., Shuman, B., Takahara, H., Toney, J., Turney, C., Urrego-Sanchez, D., Umbanhowar, C., Vandergoes, M., Vanniere, B., Vescovi, E., Walsh, M., Wang, X., Williams, N., Wilmshurst, J., and Zhang, J.: Changes in fire regimes since the Last Glacial Maximum: An assessment based on a global synthesis and analysis of charcoal data, Clim. Dynam., 30, 887–907, https://doi.org/10.1007/s00382-007-0334-x, 2008. a, b, c, d
Price, T. D.: Europe's First Farmers, Cambridge University Press, https://doi.org/10.1017/CBO9780511607851, 2000. a
Ramanathan, V. and Carmichael, G.: Global and Regional Climate Changes Due to Black Carbon, Nat. Geosci., 1, 221–227, https://doi.org/10.1038/ngeo156, 2008. a
Rubino, M., D'Onofrio, A., Seki, O., and Bendle, J.: Ice-core records of biomass burning, The Anthropocene Review, 3, 140–162, https://doi.org/10.1177/2053019615605117, 2015. a, b
Ruddiman, W.: The Anthropogenic Greenhouse Era Began Thousands of Years Ago, Clim. Change, 61, 261–293, https://doi.org/10.1023/B:CLIM.0000004577.17928.fa, 2003. a, b
Ruddiman, W. and Ellis, E.: Effect Of Per-Capita Land Use Changes On Holocene Forest Clearance And CO2 Emissions, Quaternary Sci. Rev., 28, 3011–3015, https://doi.org/10.1016/j.quascirev.2009.05.022, 2009. a
Sapart, C., Monteil, G., Prokopiou, M., Wal, R., Kaplan, J., Sperlich, P., Krumhardt, K., Veen, C., Houweling, S., Krol, M., Blunier, T., Sowers, T., Martinerie, P., Witrant, E., Dahl-Jensen, D., and Röckmann, T.: Natural and anthropogenic variations in methane sources during the past two millennia, Nature, 490, 85–88, https://doi.org/10.1038/nature11461, 2012. a, b
Schüpbach, S., Fischer, H., Bigler, M., Erhardt, T., Gfeller, G., Leuenberger, D., Mini, O., Mulvaney, R., Abram, N., Fleet, L., Frey, M., Thomas, E., Svensson, A., Dahl-Jensen, D., Kettner, E., Kjær, H., Seierstad, I., Steffensen, J., Rasmussen, S., and Wolff, E.: Greenland records of aerosol source and atmospheric lifetime changes from the Eemian to the Holocene, Nat. Commun., 9, 1476, https://doi.org/10.1038/s41467-018-03924-3, 2018. a
Simoneit, B. R. T.: A review of biomarker compounds as source indicators and tracers for air pollution, Environ. Sci. Poll. Res., 6, 159–169, https://doi.org/10.1007/BF02987621, 1999. a
Simonsen, M. F., Baccolo, G., Blunier, T., Borunda, A., Delmonte, B., Frei, R., Goldstein, S., Grinsted, A., Kjær, H. A., Sowers, T., Svensson, A., Vinther, B., Vladimirova, D., Winckler, G., Winstrup, M., and Vallelonga, P.: East Greenland ice core dust record reveals timing of Greenland ice sheet advance and retreat, Nat. Commun., 10, 4494, https://doi.org/10.1038/s41467-019-12546-2, 2019. a, b, c, d, e, f, g, h
Stein, A. F., Draxler, R. R., Rolph, G. D., Stunder, B. J. B., Cohen, M. D., and Ngan, F.: NOAA’s HYSPLIT Atmospheric Transport and Dispersion Modeling System, B. Am. Meteorol. Soc., 96, 2059–2077, https://doi.org/10.1175/BAMS-D-14-00110.1, 2015. a
Stohl, A., Berg, T., Burkhart, J. F., Fjǽraa, A. M., Forster, C., Herber, A., Hov, Ø., Lunder, C., McMillan, W. W., Oltmans, S., Shiobara, M., Simpson, D., Solberg, S., Stebel, K., Ström, J., Tørseth, K., Treffeisen, R., Virkkunen, K., and Yttri, K. E.: Arctic smoke – record high air pollution levels in the European Arctic due to agricultural fires in Eastern Europe in spring 2006, Atmos. Chem. Phys., 7, 511–534, https://doi.org/10.5194/acp-7-511-2007, 2007. a
Streeter, R., Dugmore, A., Lawson, I., Erlendsson, E., and Edwards, K.: The onset of the palaeoanthropocene in Iceland: Changes in complex natural systems, The Holocene, 25, 1662–1675, https://doi.org/10.1177/0959683615594468, 2015. a, b
Trbojević, N.: The Impact of Settlement on Woodland Resources in Viking Age Iceland, Ph.D. thesis, The School of Humanities, University of Iceland, 2016. a
Truong, C., Oudre, L., and Vayatis, N.: Selective review of offline change point detection methods, Signal Processing, 167, 107299, https://doi.org/10.1016/j.sigpro.2019.107299, 2019. a
Vannière, B., Blarquez, O., Rius, D., Doyen, E., Brücher, T., Colombaroli, D., Connor, S., Feurdean, A., Hickler, T., Kaltenrieder, P., Lemmen, C., Leys, B., Massa, C., and Olofsson, J.: 7000-year human legacy of elevation-dependent European fire regimes, Quaternary Sci. Rev., 132, 206–212, https://doi.org/10.1016/j.quascirev.2015.11.012, 2016. a
Vinther, B., Buchardt, S. L., Clausen, H., Dahl-Jensen, D., Johnsen, S., Fisher, D., Koerner, R., Raynaud, D., Lipenkov, V., Andersen, K., Blunier, T., Rasmussen, S., Steffensen, J., and Svensson, A.: Holocene thinning of the Greenland ice sheet, Nature, 461, 385–388, https://doi.org/10.1038/nature08355, 2009. a
Vinther, B. M., Clausen, H. B., Johnsen, S. J., Rasmussen, S. O., Andersen, K. K., Buchardt, S. L., Dahl-Jensen, D., Seierstad, I. K., Siggaard-Andersen, M., Steffensen, J. P., Svensson, A., Olsen, J., and Heinemeier, J.: A synchronized dating of three Greenland ice cores throughout the Holocene, J. Geophys. Res.-Atmos., 111, D13102, https://doi.org/10.1029/2005JD006921, 2006. a
Vésteinsson, O. and McGovern, T. H.: The Peopling of Iceland, Norwegian Archaeological Review, 45, 206–218, https://doi.org/10.1080/00293652.2012.721792, 2012. a
Wagner, B., Melles, M., Hahne, J., Niessen, F., and Hubberten, H.-W.: Holocene climate history of Geographical Society Ø, East Greenland – evidence from lake sediments, Palaeogeography, Palaeoclimatology, Palaeoecology, 160, 45–68, https://doi.org/10.1016/S0031-0182(00)00046-8, 2000. a, b, c, d
Warner, M.: Introduction to PySPLIT: A Python toolkit for NOAA ARL's HYSPLIT model, Comput. Sci. Eng., 20, 47–62, https://doi.org/10.1109/MCSE.2017.3301549, 2018. a
Wendl, I. A., Eichler, A., Isaksson, E., Martma, T., and Schwikowski, M.: 800-year ice-core record of nitrogen deposition in Svalbard linked to ocean productivity and biogenic emissions, Atmos. Chem. Phys., 15, 7287–7300, https://doi.org/10.5194/acp-15-7287-2015, 2015. a
Witze, A.: The Arctic is burning like never before – and that’s bad news for climate change, Nature, 585, 336–337, https://doi.org/10.1038/d41586-020-02568-y, 2020. a
You, C., Yao, T., Xu, B., Xu, C., Zhao, H., and Song, L.: Effects of sources, transport, and postdepositional processes on levoglucosan records in southeastern Tibetan glaciers, J. Geophys. Res.-Atmos., 121, 8701–8711, https://doi.org/10.1002/2016JD024904, 2016. a
Zennaro, P., Kehrwald, N., McConnell, J. R., Schüpbach, S., Maselli, O. J., Marlon, J., Vallelonga, P., Leuenberger, D., Zangrando, R., Spolaor, A., Borrotti, M., Barbaro, E., Gambaro, A., and Barbante, C.: Fire in ice: two millennia of boreal forest fire history from the Greenland NEEM ice core, Clim. Past, 10, 1905–1924, https://doi.org/10.5194/cp-10-1905-2014, 2014. a, b, c, d
Zennaro, P., Kehrwald, N., Marlon, J., Ruddiman, W. F., Brücher, T., Agostinelli, C., Dahl-Jensen, D., Zangrando, R., Gambaro, A., and Barbante, C.: Europe on fire three thousand years ago: Arson or climate?, Geophys. Res. Lett., 42, 5023–2033, https://doi.org/10.1002/2015GL064259, 2015. a, b, c, d