the Creative Commons Attribution 4.0 License.
the Creative Commons Attribution 4.0 License.
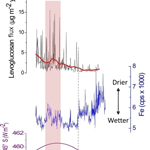
High-latitude Southern Hemisphere fire history during the mid- to late Holocene (6000–750 BP)
Natalie M. Kehrwald
Piero Zennaro
Giuseppe Pellegrino
Elena Barbaro
Roberta Zangrando
Xanthi X. Pedeli
Cristiano Varin
Andrea Spolaor
Paul T. Vallelonga
Andrea Gambaro
Carlo Barbante
We determined the specific biomass burning biomarker levoglucosan in an ice core from the TALos Dome Ice CorE drilling project (TALDICE) during the mid- to late Holocene (6000–750 BP). The levoglucosan record is characterized by a long-term increase with higher rates starting at ∼ 4000 BP and peaks between 2500 and 1500 BP. The anomalous increase in levoglucosan centered at ∼ 2000 BP is consistent with other Antarctic biomass burning records. Multiple atmospheric phenomena affect the coastal Antarctic Talos Dome drilling site, where the Southern Annular Mode (SAM) is the most prominent as the Southern Annular Mode Index (SAMA) correlates with stable isotopes in precipitation throughout the most recent 1000 years of the ice core. If this connection remains throughout the mid- to late Holocene, then our results demonstrate that changes in biomass burning, rather than changes in atmospheric transport, are the major influence on the TALDICE levoglucosan record. Comparisons with charcoal syntheses help evaluate fire sources, showing a greater contribution from southern South American fires than from Australian biomass burning. The levoglucosan peak centered at ∼ 2000 BP occurs during a cool period throughout the Southern Hemisphere, yet during a time of increased fire activity in both northern and southern Patagonia. This peak in biomass burning is influenced by increased vegetation in southern South America from a preceding humid period, in which the vegetation desiccated during the following cool, dry period. The Talos Dome ice core record from 6000 to ∼ 750 BP currently does not provide clear evidence that the fire record may be strongly affected by anthropogenic activities during the mid- to late Holocene, although we cannot exclude at least a partial influence.
- Article
(3728 KB) - Full-text XML
-
Supplement
(82 KB) - BibTeX
- EndNote
Fire and climate reciprocally influence one another. Biomass burning affects the chemical composition of the atmosphere, the global carbon cycle and the radiative balance due to the emission of greenhouse gasses (carbon dioxide, carbon monoxide, methane and nitrous oxide) as well as aerosols (Andreae and Merlet, 2001; Akagi et al., 2011; Bowman et al., 2009; Keywood et al., 2013; Galanter et al., 2000; van der Werf et al., 2004; Harrison et al., 2010). Temperature, precipitation and atmospheric carbon dioxide (CO2) control both fuel productivity and flammability where biomass growth, fire ignition and spread are favored by the oscillation of wet and dry conditions (Westerling et al., 2006; Daniau et al., 2010; Marlon et al., 2013; Pyne, 2001; Rollins et al., 2002). Spatial variability in climate and the resulting fire–vegetation–climate interactions further complicate fire dynamics (Lynch et al., 2004). For example, Holocene fire records from the same region may not be synchronous over centennial to millennial timescales (Brunelle and Whitlock, 2003). Furthermore, regional anthropogenic deforestation for the creation of open spaces for croplands and herding adds another variable to the fire–climate system. Anthropogenic impacts complicate fire dynamics, as humans are able to both provoke and extinguish fires (Clare-Smith et al., 2016; Zohary et al., 2012; Tauger, 2011; Ruddiman, 2003; Marlon et al., 2008; Power et al., 2008; Chuvieco et al., 2008; Archibal et al., 2009). The early anthropogenic hypothesis proposed by Ruddiman (2003) is still debated in terms of the scale of the effect of early agriculture on the global climate system, but there is no doubt that land use changes affect climate at regional scales (Broeker and Stocker, 2006; Joos et al., 2004; Singarayer et al., 2011; Mitchell et al., 2013; Kaplan et al., 2009, 2011).
Charcoal is an established proxy for reconstructing past fire regimes from sedimentary archives. Distinguishing microscopic (< 100 µm) from macroscopic (> 200 µm) charcoal allows the reconstruction of fire history at regional and local scales, respectively (Clark et al., 1996; Marlon et al., 2013; Carcaillet et al., 2002; Whitlock et al., 2007). The Global Charcoal Database (GCD) (Power et al., 2010, http://www.paleofire.org/; last access: 11 July 2017) provides a useful dataset for sedimentary research records of fire (Blarquez et al., 2014; Marlon et al., 2008, 2013; Power et al., 2008, 2013; Daniau et al., 2012; Mooney et al., 2011; Vanniere et al., 2011) and the paleofire R package creates customized charcoal syntheses (https://github.com/paleofire/GCD; last access: 12 November 2017, Blarquez et al., 2014). Although the recent Global Charcoal Database version 3 (GCDv3) compiles more than 700 charcoal records (Marlon et al., 2016), the sites included in GCD are not homogenously distributed, due in large part to the location of lakes, leading to geographical over- or underrepresentation.The atmospheric transport of macrocharcoal only extends a few kilometers (Carcaillet et al., 2001) and therefore charcoal records cannot encompass some areas such as deserts or polar regions.
Ice cores complement charcoal syntheses as they can reconstruct fire histories at regional to semi-hemispheric scales (Legrand et al., 1992). Fire proxies in ice cores include inorganic chemicals such as ammonium, nitrate and potassium (Legrand et al., 2016), black carbon (BC; McConnel, 2007), and organic compounds such as formate, oxalate, phenolic compounds and levoglucosan (Legrand et al., 2016; Zangrando et al., 2013, 2016). These proxies originate from biomass burning, but not all of these materials are exclusively emitted by combustion. Several organic compounds are strongly related to the type of fuel, for which, for example, conifer combustion produces dehydroabietic acid (Fine et al., 2002). However, other proxies such as oxalate, phenolic compounds and potassium are not specific indicators of biomass burning. Emission factors and lifetimes differ among proxies, and atmospheric transport between the source region and the drilling site may influence individual records (Rubino et al., 2016).
Levoglucosan (1,6- anhydro β-D-glucopyranose) is a source-specific proxy for fire that is produced by cellulose and emitted by biomass burning, with a maximum yield centered around ∼ 250 ∘C (Kuo et al., 2008). The atmospheric lifetime of levoglucosan is actively debated with estimates ranging between a few days (Hoffman et al., 2010; Hennigan et al., 2010) and a few weeks (Bai et al., 2013; Slade and Knopf, 2013). Ice core levoglucosan reconstructions currently provide biomass burning histories from Northern Hemisphere locations. Levoglucosan records extending back 15 000 years reconstruct high-northern-latitude fire history in Greenland (Zennaro et al., 2015). Mountain glacier levoglucosan records depict regional fire histories in Kamchatka and the Tibetan Plateau (Kawamura et al., 2012; Yao et al., 2013). However, Southern Hemisphere levoglucosan records still leave much to be explored. Antarctic levoglucosan records will likely differ from Arctic records due to the substantially smaller land masses surrounding the ice sheet and the long distances required for atmospheric transport of biomass burning material. The southernmost tip of Patagonia, the closest continental landmass to Antarctica, only extends to ∼ 55∘ S, whereas the Arctic contains the largest land masses in the world. Although long-term levoglucosan records have never been reported for Antarctic ice cores, several studies determine other biomass burning by-products (i.e., secondary organic aerosol and BC) for the last few decades and centuries (Wolff and Cachier, 1998; Fiebig et al., 2009; Hara et al., 2010; Hu et al., 2013; Weller et al., 2013; Pasteris et al., 2014) as well as for the Holocene (Arienzo et al., 2017).
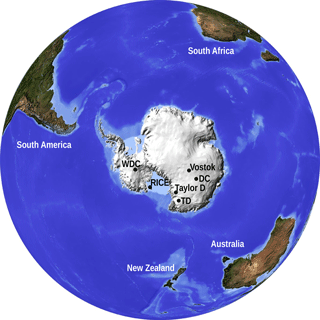
Figure 1Map of the Antarctic region including the Talos Dome (TD), Vostok, Taylor Dome (Taylor D), Dome C (DC), RICE and WAIS Divide ice core sites mentioned in this paper.
Hemispheric fire history since the Last Glacial Maximum, derived from marine records (Daniau et al., 2012), demonstrates relatively high fire activity during the Holocene where biomass burning is more pronounced at high latitudes. The Northern Hemisphere and Southern Hemisphere fire histories substantially differ from one another (Daniau et al., 2012), where the Southern Hemisphere fire history is characterized by a widespread spatial heterogeneity (Power et al., 2008). From the mid- to late Holocene onward, sea levels approached near-modern levels, and most regional Southern Hemisphere glacial ice had melted. The increase in human population in Australasia and South America and the associated shifting vegetation types and/or the strengthening of the El Niño–Southern Oscillation (ENSO) activity explain the heterogeneity of fire patterns since 3000 BP (Power et al., 2008; Whitlock et al., 2007).
We aim to reconstruct Southern Hemisphere fire history by determining levoglucosan concentration in an ice core obtained from the TALos Dome Ice CorE drilling project (TALDICE) during the mid- to late Holocene (6000–750 BP). Talos Dome (159∘11′ E, 72∘49′ S, 2315 m a.s.l.; Fig. 1) is located in the South Pacific/Ross Sea sector of the East Antarctic Plateau (https://www.taldice.org; last access: 7 December 2017). The relatively high snow accumulation rates (80 kg m−2 yr−1, average 2004–1259 AD; Stenni et al., 2002) enable accurate dating of the core (Buiron et al., 2011; Veres et al., 2013) and high-resolution climate analyses during the Holocene (Albani et al., 2012a; Delmonte et al., 2013). Charcoal syntheses provide information of biomass burning source regions and changing fire activity. These fire and climate records can provide a synthesized history of high-latitude Southern Hemisphere biomass burning in a relatively stable climate regime that is subject to an increasing human influence.
2.1 Ice core samples and analysis
We analyzed 266 ice core samples from the TALDICE (TD) ice core, covering the depth interval between 90 and 403 m. Each sample represents the uppermost 15 cm of a 1 m ice core section. These sections were the ice remaining after other analyses performed by Italian TALDICE researchers. The samples are therefore discontinuous and equidistant in space but not in time. The samples thus have the potential to miss fire peaks due to their discontinuous nature. The ice core samples were stored at Ca' Foscari University of Venice at −20 ∘C until analysis. Before the analysis, the ice samples were decontaminated by washing the outermost section three times using ultrapure water (Elga LabWater, Marlow, UK) in an ISO 5 clean room in order to remove possible drilling fluid residuals or impurities. During the washing, the samples were held using PTFE tongs (Nalgene Corporation, Rochester, NY) previously immersed for 2 days in 5 and 1 % HNO3 solutions and rinsed with ultrapure water. The same procedure was used for decontaminating low-density polyethylene (LDPE) bottles and vials. Ice samples were melted at room temperature in 125 mL LDPE bottles and then transferred to 15 mL LDPE bottles and stored at −20 ∘C in triple polyethylene bags. These precautions were necessary for minimizing any possible contamination of the samples.
Levoglucosan analysis was performed using a method specifically developed for the analysis of polar ice samples (Gambaro et al., 2008, and as modified in Zennaro et al., 2014, 2015) based on liquid chromatography coupled with a triple quadrupole mass spectrometer (HPLC/(-)ESI-MS/MS). Briefly, we injected 300 µL of the sample in a HPLC system (Agilent 1100, Waldbronn, Germany). Chromatographic separation was obtained using a C18 Synergi Hydro column (4.6 mm i.d., 50 mm length, 4 µm size particles; Phenomenex, Torrance, CA) and an autosampler was equipped with a LOOP Multidraw 44 Upgrade Kit G1313-68711. The samples were eluted with methanol (ultragradient, H411, Romil Ltd, Cambridge, UK) and ultrapure water (18.2 MΩ, TOC 1 ppb, PURELAB Pulse and PURELAB Flex, Elga). The mass spectrometer was an API 4000 (Applied Biosystems/MDS SCIEX, Toronto, Ontario, Canada) equipped with an ion spray source ((-)ESI) Turbo V operating in negative polarity. Mass ∕ charge (m∕z) ratios used for the quantification were 161 and 113 for levoglucosan and 167 and 118 for 13C-labeled levoglucosan.
This method limits pre-analytical procedures that may affect the quantitative determination and the sample contamination and it allows the analysis of levoglucosan at levels of a few picograms per milliliter. We quantified the results using isotopic dilution (13C6-labeled levoglucosan as an internal standard; Cambridge Isotope Laboratories Inc., Andover, MA) and instrumental response factors were determined prior, during and after each set of analyses in order to evaluate eventual deviation from the instrumental response. The instrumental limit of quantification (LoQ) for levoglucosan was 4 pg mL−1, determined following the analytical method reported in Gambaro et al. (2008) and as modified in Zennaro et al. (2014). From an analytical point of view, a reliable procedural LoQ is difficult to determine in this case, due to the lack of a suitable aqueous matrix. The glacier water often contains lower concentrations of levoglucosan than the ultrapure laboratory water, thereby complicating obtaining a true LoQ. However, as this method only requires as a few pre-analytical procedures, for which these steps are always performed in a dedicated clean room, we therefore use the instrumental values as the LoQ. Levoglucosan concentrations and their associated errors are reported in the Supplement (see the file rawdata.xls)
2.2 Dating and data treatment
The ice core sample ages were calculated using the AICC2012 age scale (Bazin et al., 2013; Veres et al., 2013). The depth–age model and associated errors are also reported in the Supplement (rawdata.xls). Using this depth–age model, we normalized levoglucosan concentrations into flux values by dividing the concentrations by the accumulation rate for each corresponding ice section (Buiron et al., 2011).
Charcoal syntheses were performed by using the paleofire R package associated with the Global Charcoal Database by extracting a 20-year bin time window (consistent with the time period covered by each levoglucosan sample) from 6000 BP to present. Detection and separation of anomalous intense peaks from the levoglucosan series were based on Cook's distance (Cook, 1977), after generalized additive modeling (GAM) of the relationship between each series and age using the R package mgcv (Wood, 2006). Preliminary data analysis revealed that levoglucosan records were highly skewed, and we therefore log-transformed all data to reduce skewness before any further analysis. Potential associations between levoglucosan and atmospheric transport indicators as well as fire sources and climate parameters were analyzed using a slot correlation with Gaussian kernel to account for the irregularly sampled data (Rehfeld et al., 2011). In order to investigate the statistical significance of the resulting correlation estimates (r), we constructed 95 % bias-corrected and bias-accelerated (BCa) confidence intervals based on 5000 bootstrap samples. Calculations were made in R (R Core Team, 2017) using the boot package (Canty and Ripley, 2017; Davison and Hinkley, 1997).
3.1 Levoglucosan in the Talos Dome ice core
In the 266 TD ice core samples analyzed in this work, levoglucosan concentrations ranged from 4 (corresponding to the LoQ) to 1100 pg mL−1. However, in 68 of the 266 samples the levoglucosan concentrations were below the LoQ. The highest concentrations were lower, but of the same order of magnitude, than the major levoglucosan peaks detected in the NEEM (Greenland) ice core (185–1767 pg mL−1) during the late Holocene (Zennaro et al., 2014) and in the Ushkovsky (Kamchatka Peninsula) ice cap (10–5000 pg mL−1) during the last 3 centuries (Kawamura et al., 2012). Levoglucosan concentrations from these remote regions are significantly lower than in environmental archives closer to more human-influenced areas such as in the Muztagh Ata and Tanggula ice cores where concentrations were 10–718 and 10–93 ng mL−1, respectively (Yao et al., 2013).
The complete mid- to late Holocene Talos Dome levoglucosan record is shown in Fig. 2b. Levoglucosan spikes potentially indicate extreme fire events, more frequent fire activity during the short temporal interval, and/or more efficient atmospheric transport during the fire event. These anomalous intense peaks significantly affect the temporal trend in the record even when adopting different smoothing approaches (Zennaro et al., 2014). In order to better identify which individual peaks could influence the entire dataset, we used Cook's distance to indicate outliers (n=9), which resulted in peaks with values greater than ∼ 16 µg m−2 yr−1 (Fig. 2b). We therefore separate these intense peaks from the longer-term fire history (Fig. 2c).
The levoglucosan flux is lowest during the oldest part of the record (between 6000 and 4000 BP), while a significant long-term increase occurs after approximately 4000–3500 BP (Fig. 2). Levoglucosan increases between ∼ 2250 and ∼ 2000 BP and majorly increases between ∼ 1250 and ∼ 800 BP. The timing of the individual levoglucosan spikes roughly occur between 2200–1800 and 1200–900 BP, corresponding with the major long-term increases. Thus, these extreme peaks should be considered as part of a more widespread period when levoglucosan was generally higher in the Talos Dome mid- to late Holocene sequence rather than isolated fire events.
The stability and atmospheric transport dynamics of levoglucosan in the atmosphere may influence the record. OH• radicals, which can occur in the atmosphere in both the gas and aqueous phases, are able to oxidize levoglucosan, leading to degradation during transport (Hennigan et al., 2010; Hoffman et al., 2010). The extent of this degradation is currently unknown as recent studies also propose that levoglucosan can exist in the atmosphere for up to 26 days (Bai et al., 2013). The atmospheric stability of levoglucosan and atmospheric transport dynamics both influence fire signals in polar ice (Zennaro et al., 2014; Legrand et al., 2016). Although large amounts of levoglucosan are emitted during biomass burning and are quantifiable even at remote distances from the source (Zennaro et al., 2014, 2015; Kehrwald et al., 2012), the detected levoglucosan concentrations may be affected by a combination of transport and degradation, thus making the signal over- or underrepresentative of the initial individual fire event. Conditions such as varying precipitation amounts, dry versus wet deposition and the distance from the fire source can modulate the levoglucosan signal, thus resulting in a reduction of the effects of megafire events or amplifying modest fires. However, the stability of levoglucosan once trapped in the ice may be less significant. Levoglucosan profiles in polar ice cores do not result from simple degradation curves, supporting the assumption of the stability of this proxy in ice cores (Zennaro et al., 2014, 2015; Kehrwald et al., 2012).
The attribution of the levoglucosan spikes to individual large fire events is difficult to assess as atmospheric transport and stability may alter the signal, resulting in an amplification of modest fires. A similar situation occurs for individual peaks in charcoal records as these spikes can either be intense local fire events or can result from an increase in short-term transport. For example, the high charcoal signals observed in Laguna Padre Laguna (Argentina) between ∼ 2000 and ∼ 1500 BP and at Laguna Zeta (Argentina) between ∼ 2500 and ∼ 2000 BP (Iglesias and Whitlock, 2014) are consistent with the spikes observed in the levoglucosan record, as well as intense fire episodes recorded between ∼ 2300 and ∼ 2100 BP in the Wingecarribee Swamp (southern Australia; de Montford, 2008; ID site = 857 in the GCD) and at 2160 BP in Eweburn Bog (New Zealand; Ogden et al., 1998; ID site = 441 in the GCD). A possible correspondence with levoglucosan and these individual charcoal spikes is only speculative. Comparing trends in long-term fire activity, as identified by smoothed levoglucosan records and charcoal syntheses, is more indicative of changes in biomass burning as opposed to comparing individual, likely localized, events. We therefore only discuss biomass burning trends rather than individual spikes from this point forward.
3.2 Atmospheric transport
Unlike central East Antarctic Plateau locations (i.e., Vostok Petit et al., 1999, and EPICA Dome C (EDC) Lambert et al., 2008), Talos Dome is significantly influenced by dust of local origin. Talos Dome contains dust grains larger than 5 µm (diameter) that are absent in the EDC core (Delmonte et al., 2010a). The finer dust fraction (1–5 µm) at Talos Dome is considered to be a mixture of local and remote sources, while this same fraction in EDC is due to long-range transport for which the net dust amount is substantially less than at Talos Dome. However, a clear declining trend in dust flux since ∼ 8000 BP was evident in both ice core records (Albani et al., 2012a; Delmonte et al., 2005). The progressive opening of the Ross Sea embayment from the early Holocene onward increased the relative contribution of regional dust (Hall et al., 2009; Stenni et al., 2011; Masson-Delmotte et al., 2011; Albani et al., 2012 a).
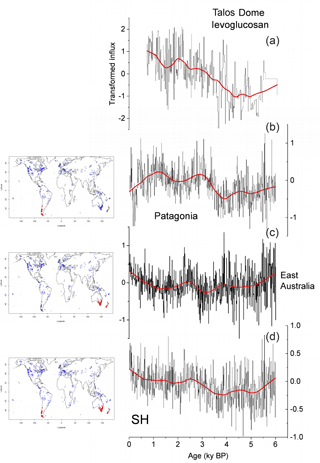
Figure 3Box–Cox-transformed levoglucosan influx and charcoal synthesis (bins = 20 years) from Patagonia, eastern Australia and Southern Hemisphere synthesis from the GCD. LOWESS smoothing (in red) with SPAN parameters 0.2. Red dots on the map indicate the location of the charcoal records.
The composition of mineral dust provides useful indications of the original source and subsequent atmospheric transport. The isotopic composition of dust in central East Antarctica suggests that southern South America was the primary dust source during glacial periods, with possible contributions from Australia (Delmonte et al., 2008, 2010b; Grousset et al., 1992; Basile et al., 1997; Gabrielli et al., 2010; Vallelonga et al., 2010). During the Holocene, the considerable contribution of dust from proximal ice-free areas of northern Victoria Land, Antarctica, located at a similar altitude with respect to the drilling site (Delmonte et al., 2010a), renders the isotopic analysis of Talos Dome dust unreliable. Nevertheless, the 87Sr ∕ 86Sr vs. 143Nd ∕ 144Nd isotopic signature of mineral dust at other East Antarctica sites (Vostok and Dome C) prompted Revel-Rolland (2006) to consider a possible eastern Australian provenience. Although the southern South American source was reduced with respect to glacial periods, several authors proposed that the South American provenance also persisted during the interglacials (Holocene and MIS-5), but where the source shifted to lower-latitude regions (Delmonte et al., 2008; Gaiero et al., 2007). This hypothesis is also supported by the lithium enrichment factors from the EDC ice core that are similar to continental ice cores from Bolivia (Siggaard-Anderses et al., 2007; Correia et al., 2003) and by 10-day back trajectory plots that showed a South American source for organic carbon in East Antarctica (Antony et al., 2014). However, a relative weakening of the South American source during interglacials cannot be excluded (Delmonte et al., 2007, 2008; Gaiero et al., 2007) with a feasible combination of both Australian and South American contributions during the Holocene.
We cannot stress strongly enough that the source regions and atmospheric transport of mineral dust and organic materials in smoke plumes may not be the same. Comparisons between Talos Dome levoglucosan (log transformed) and mineral dust < 5 µm (log-transformed data from Albani et al., 2012) provide a statistically significant negative correlation (r (95 % CI) = −0.215 (−0.365, −0.103)). These results suggest that at least part of the levoglucosan record at Talos Dome may be affected by a different circulation pathway than that of the mineral dust.
3.3 Biomass burning sources
The levoglucosan profile likely reflects fire activity occurring in the nearby continents, as Antarctica contains essentially no biomass available for burning. Although charcoal is a local proxy for fire (Whitlock and Larsen 2002; Clark et al., 1996, 1988), synthesizing individual records from the GCD (Marlon et al., 2016; Blarquez et al., 2014) is a useful tool for reconstructing fire history across regions (Marlon et al., 2013, 2016). We compiled charcoal syntheses from the following potential source areas of the fire signal recorded in the Talos Dome ice core: (i) eastern Australia (including New Zealand) between 90 and 30∘ S (latitude) and between 160 and 180∘ E (longitude), (ii) southern South America between 90 and 30∘ S (latitude) and between 60 and 80∘ W (longitude), and (iii) the Southern Hemisphere covering areas between 90 and 30∘ S (Fig. 3). The number of charcoal records varies by region where the eastern Australia and southern South America composite records were obtained by combining n=131 and n=25 sites, respectively. In order to compare the levoglucosan record with the charcoal syntheses, we applied a Box–Cox transformation to the records (Power et al., 2008). As is evident from Fig. 3, the levoglucosan increase observed at ∼ 3500 BP coincides with the same increase in southern South America and Southern Hemisphere charcoal syntheses. Conversely, the levoglucosan increase centered at ∼ 2000 BP, which also includes more frequent outliers, is not supported by any charcoal records. The correlation analysis of Talos Dome levoglucosan (log transformed; outliers excluded) and charcoal records produces a statistically significant positive correlation with southern South America (r (95 % CI) = 0.333 (0.242, 0.438)) but not in eastern Australia (r (95 % CI) = 0.011 (−0.078, 0.095)). Levoglucosan does positively correlate with the total SH charcoal synthesis (r (95 % CI) = 0.225 (0.138,0.317)) over the investigated time period of 750–6000 BP.
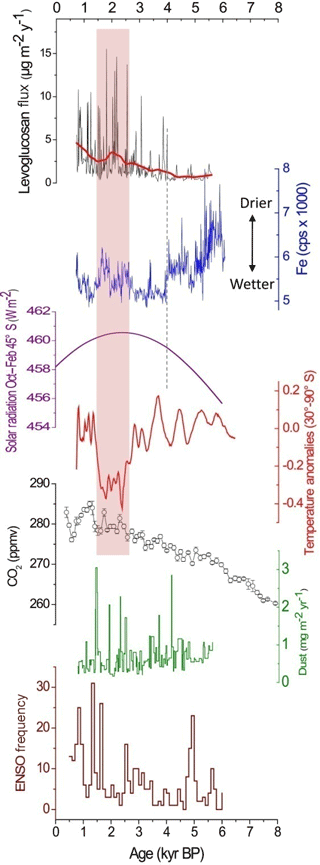
Figure 4Levoglucosan flux and Fe content in marine sediments from southern Chile (from Lamy et al., 2001); October–February 45∘ S solar radiation (from Laskar et al., 2004), temperature anomalies between 30 and 90∘ S (Marcott et al., 2013), CO2 from Taylor Dome (Indermühle et al., 1999), dust from Talos Dome (Albani et al., 2012) and ENSO 100-year frequency (Moy et al., 2002).
Talos Dome levoglucosan and southern South America charcoal records (Fig. 3) both depict an increasing long-term trend in biomass burning from 4000 to 750 BP, although these trends do not perfectly coincide. These records differ both before and after these ∼ 3000 years of similarity. The Talos Dome record begins its major and sustained increase in fire activity around 4500 BP, while the southern South American record decreases during this time period. The southern South America and eastern Australian records substantially diverge after 750 BP, when the southern South American fires decrease and the eastern Australian and SH fires both markedly increase. The Talos Dome levoglucosan record does not extend to the present, and so comparisons with this major difference in regional fire sources are not possible.
These results may suggest a higher contribution from southern South American fires rather than Australian biomass burning over centennial to millennial timescales between 4000 and 750 BP, although the contribution from eastern Australia cannot be completely neglected. However, it is also possible that this observation may be due to the higher regional variability in eastern Australian fire activity (Mooney et al., 2011). During the mid-Holocene, sites in the far south of southeastern Australia showed higher fire activity due to a shift toward more moisture-stressed vegetation, while sites in the Southern Tablelands and east of the Great Dividing Range showed less fire activity with the region supporting relatively moisture-demanding vegetation (Pickett et al., 2004; Mooney et al., 2011). Thus, over a wider spatial scale, these contributions tend to cancel one another out. As a result, although the southeastern Australia charcoal synthesis is characterized by higher values at about 6000 BP, during the mid-Holocene, only small local increases at ∼ 4000 and ∼ 2500 BP were observed. The southern South American fire synthesis is generally more homogenous, although some regional spatial variability between northern and southern Patagonia does exist (Mundo et al., 2017). The southern South American region defined in these charcoal syntheses extends from Patagonia to the Argentinian Pampas, encompassing grassland, steppe, desert and forest vegetation.
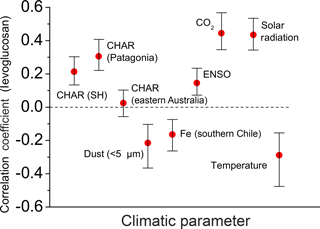
Figure 5Slot correlation with 95 % bootstrap confidence interval between levoglucosan recorded at Talos Dome (log transformed) and (i) charcoal synthesis (CHAR) from Patagonia, (ii) eastern Australia, (iii) the Southern Hemisphere, (iv) dust particles at Talos Dome (from Albani et al., 2012), (v) iron record from southern Chile (Lamy et al., 2001), (vi) carbon dioxide from Taylor Dome (Indermühle et al., 1999), (vii) ENSO 100-year frequency (Moy et al., 2002), (viii) October–February 45∘ S solar radiation (from Laskar et al., 2004), and (ix) temperature anomalies between 30 and 90∘ S (Marcott et al., 2013).
3.4 Drivers of biomass burning
The iron content in southern Chile marine sediments reconstructs rainfall changes due to latitudinal shifts of the Southern Westerlies during the Holocene (Lamy et al., 2001; see also Fig. 4 in this paper). This reconstruction is consistent with regional terrestrial paleoclimate datasets (Villagran et al., 1990; Lamy et al., 2001) and indicates more arid conditions from 7700 to 4000 BP, followed by a general increase in rainfall from 4000 BP to the present day. This finding is consistent with the hypothesis that fire activity in southern South America is mainly driven by fuel availability (Iglesias and Whitlock, 2014; Iglesias et al., 2014). However, the main drivers of fire may vary within Patagonia as fire activity in southern Patagonia depends upon fuel desiccation due to droughts rather than the years of wet climate conditions that result in more vegetation, which is an important driver of fire in northern Patagonia (Mundo et al., 2017). The southern South American precipitation record (Lamy et al., 2001) and Talos Dome levoglucosan record demonstrate a relationship between rainfall and fire activity in the mid- to late Holocene (Fig. 4). Bivariate analysis indicates a significant negative correlation between levoglucosan (log transformed; outliers excluded) and iron records (log transformed) (r (95 % CI) = −0.183 (−0.277, −0.083)), suggesting that when arid conditions characterized southern South America, the levoglucosan signal was low and started to increase when the Southern Westerlies strengthened and shifted equatorward, bringing more humid conditions to the continent. This interpretation is consistent with the increase in more humid conditions in continental records (Iglesias and Whitlock, 2014; Iglesias et al., 2014), although it cannot be excluded that the changes in the westerly wind belt may have increased, at least in part, the transport of aerosols to Antarctica.
Southern Hemisphere temperature (r (95 % CI) = −0.272 (−0.428, −0.147); see also Fig. 5) substantially decreases between ∼ 2500 and ∼ 1500 BP, where this decrease is not evident in either the solar irradiance or greenhouse gas concentrations (Fig. 4). Carbon dioxide increases fairly steadily from the mid- to late Holocene onward in the Taylor Dome ice core (Indermühle et al., 1999; Fig. 4), while the general levoglucosan trend increases beginning at ∼ 4000 BP, yet includes a major dip in fire activity around ∼ 2800 BP (r (95 % CI) = 0.455 (0.357 0.576)). This difference is to be expected, as CO2 in ice cores represents a globally mixed signal while levoglucosan in polar ice cores is a regional to semi-hemispheric signal (e.g., Zennaro et al., 2014, 2015). Strong ENSO events and periods affect high-latitude Southern Hemisphere climate by increasing mean Southern Hemisphere air temperature (Schneider and Steig, 2008), expanding sea ice east of the Antarctic Peninsula (Mayewski et al., 2017, and references therein) and delivering snowfall to East Antarctica (Roberts et al., 2015). The strengthening of ENSO beginning around ∼ 5000 BP increased short-term fluctuations in Patagonian vegetation (Moy et al., 2002; Iglesias et al., 2014) and increased precipitation near northern Patagonia as evidenced by the iron record in marine sediments (Lamy et al., 2001; Lamy et al., 2004). The iron record and changes in ENSO strength (log transformed) also correlate with the Talos Dome levoglucosan record (log transformed) (r (95 % CI) = 0.172 (0.092, 0.354)), although to a lesser extent than solar radiation and greenhouse gases. ENSO variability has a weak teleconnection with fire activity (Bisiaux et al., 2012; Mundo et al., 2017), for which the weakness of this connection can be ascribed to the regional nature of ENSO with a greater ENSO influence on northern rather than southern Patagonia.
Variable and generally wet conditions prevailed in South America after 4000 BP, resulting in more available biomass and increased fire events. However, biomass burning increased even more when, at ∼ 2500 BP, Patagonia became drier yet still contained an abundance of vegetation from the previous humid period (Iglesias et al., 2014). This 1000-year peak in levoglucosan (between 2450 ± 100 and 1600 ± 100 BP) occurs at the same time as a local decrease in temperature between 2500 and 1500 BP. As the vegetation from the previous humid period would not likely remain for almost 1000 years, and as it is unlikely that decreased temperatures triggered an increase in fire activity, we propose three possible explanations: (i) precipitation–evaporation balance and/or aerosol feedbacks, (ii) different atmospheric transport and (iii) influence of insolation.
- i.
Studies of long-term fire activity variations since the Last Glacial Maximum and through the Holocene demonstrate that temperature is the main driver of fire activity over a global scale, while the precipitation–evaporation (P–E) balance is the second-most important factor (Daniau et al., 2012). The net effect of both temperature and P–E strongly depend on the initial conditions and fuel availability. Fire does not respond linearly to temperature, and an increase in temperature does not directly result in more fire under warm rather than cold conditions (Daniau et al., 2012). The relation between fire and P–E is unimodal, meaning an increase in P–E under dry or moist conditions leads to an increase or decrease in fire, respectively. This long-term observation contrasts with our record, in which an increase in fire activity coincides with a net decrease in temperature. It must be noted, however, that our observations do not represent a global signature and the relatively cold period was limited to only approximately 1000 years in the framework of the current interglacial. Moreover, fires emit large quantities of both CO2 and particulates. While CO2 warms the atmosphere, many aerosols can decrease temperature by shielding the solar radiation that reaches the surface. As a net result, at least over local to regional scales, aerosol emissions lead to a cooling effect, as observed in the Kuwait oil fires, where a reduction in ground-level temperature resulted from the absorption of solar radiation by the smoke (Bakan et al., 1991). However, although aerosol emissions can impact the surface temperature, this hypothesis is very difficult to support for a time period of ∼ 1000 years and over such a large spatial scale.
- ii.
Stacked SH proxy data between 30 and 90∘ S demonstrate a major temperature decrease between 2500 and 1500 BP (Fig. 4; Marcott et al., 2013). During this time period, the Talos Dome, West Antarctic Ice Sheet Divide (WAIS Divide) and the Roosevelt Island Climate Evolution (RICE) ice core isotope and snow accumulation records positively correlate (Bertler et al., 2018), showing that decreased temperatures are associated with less accumulation and vice versa. This correlation between temperature and accumulation applies to each site, yet during this time period, the stable isotopes of the RICE ice core in the eastern Ross Sea and Talos Dome in the western Ross Sea have phases when they differ from one another, creating a distinct Ross Sea dipole. During the time period of increased fire activity in the Talos Dome ice core ∼ 2500 to ∼ 1500 BP, the following relationships existed between detrended, normalized stable isotopes in Talos Dome and the RICE ice core: ∼ 2500 to ∼ 2150 BP in phase, ∼ 2150 to ∼ 1750 BP out of phase and ∼ 1750 to 1100 BP in phase (Bertler et al., 2018). This difference suggests that the air mass trajectories affecting these sites were similar when the records were in phase, but diverged when the ice core records were out of phase. In general, this anticorrelation between RICE and Talos Dome is strongest when the Southern Annular Mode Index (SAMA) is negative (Abram et al., 2014). The SAM is defined as the difference in zonal mean atmospheric pressure between ∼ 40 and ∼ 65∘ S (Marshall, 2003). This atmospheric link between midlatitudes and Antarctica leads to cooler, drier conditions in East Antarctica and warmer, wetter conditions in West Antarctica when the SAM index is positive (Marshall and Thompson, 2016). A positive SAM is associated with a southward shift of storm tracks over South America, and possibly increased transport of aerosols from South America to Antarctica (Christie et al., 2011; Abram et al., 2014). Over the 1000 years of the quantified SAMA, the Talos Dome stable isotopes and SAMA have remarkably similar patterns and timing where the positive SAMA coincides with increased temperatures deduced from the ice core stable isotope record (Bertler et al., 2018). The coastal location of Talos Dome is not entirely influenced by the teleconnections impacting West and East Antarctica (Bertler et al., 2018) and so this strong correspondence between SAMA and the Talos Dome stable isotopes is surprising. If this connection between SAMA and stable isotopes in precipitation near Talos Dome remains the same back to 2500 BP, then the 1000-year peak in levoglucosan (between 2450 ± 100 and 1600 ± 100 BP) encompasses both positive and negative SAM periods. Therefore, changes in atmospheric transport may not be the primary cause of the increased biomass burning in Talos Dome.
- iii.
The Patagonian fire season occurs during the austral spring and summer when major fire years coincide with severe droughts in December (Mundo et al., 2017). Arienzo et al. (2017) evaluated the difference in insolation during the growing (February) and burning season (October) at 15∘ S, with a positive correlation with the BC record in Antarctica, during the entire Holocene. BC is produced by both fossil fuel and biomass burning, and the dominant preindustrial source is fire activity. Therefore, insolation may influence both the burning and growing seasons. Fire occurrence in Patagonia is influenced prevalently by the growing season in the northern regions and prevalently by the summer droughts in the southern (Mundo et al., 2017). As different drivers affect fires in Patagonia, we opted to consider the insolation during the period between October and February to take into account to the overall contributions. A significant positive correlation with solar radiation in October–February at 45∘ S (from Laskar et al., 2004) (r (95 % CI) = 0.378 (0.280, 0.494); see also Fig. 5) is depicted by a long-term increase up to approximately 2500 BP. The cooling period centered at about 2000 BP coincides with maximum values of October–February solar radiation and a shift to drier conditions in South America, characterized by initial conditions with available fuel, thus enhancing biomass burning in Patagonia in this particular time period.
Although the levoglucosan record is consistent with October–February insolation at 45∘ S, our levoglucosan results differ from the insolation at 15∘ S used by Arienzo et al. (2017) to depict the growing and burning seasons in South American regions, potentially influencing fire histories in Antarctic ice cores. This difference may be due to the atmospheric lifetimes and/or deposition of the two proxies. As previously mentioned, studies of the atmospheric lifetime of levoglucosan range between a few days (Hoffman et al., 2010; Hennigan et al., 2010) and a few weeks (Bai et al., 2013; Slade and Knopf, 2013), and levoglucosan originating at 15∘ S may not reach Antarctica. However, combining ice core BC with multiple climate model simulations results in BC lifetimes ranging from 3.9 to 15.2 days (Lee et al., 2012), with 4 days as a reasonable BC lifetime under present atmospheric conditions (Bauer et al., 2013), suggesting that 15∘ S is also a distant source for BC. As BC is primarily deposited through wet deposition (Flanner et al., 2007), changes in hydroclimate primarily affect BC deposition in Antarctica (Arienzo et al., 2017). However, as levoglucosan is deposited by both wet and dry deposition, changes in the hydroclimate are not the only possible influence on levoglucosan flux (Bertler et al., 2018; Zennaro et al., 2015).
Arienzo et al. (2017) argue that BC in Antarctica reflects Southern Hemisphere biomass burning rather than atmospheric transport as BC is well mixed in the Antarctic atmosphere. BC concentration and flux are influenced by the amount of precipitation as BC is deposited through wet deposition (Flanner et al., 2007). A continuous record of BC and ammonium from the coastal East Antarctic B40 ice core (see Fig. 1) demonstrates a peak in biomass burning centered around ∼ 2000 BP, which is consistent with our results. One of the strengths of BC is that it can be continuously determined at high resolution in unbroken ice. However, the brittle ice section of the WAIS Divide ice core results in a semicontinuous record of BC and ammonium from ∼ 6000 BP to the present (Arienzo et al., 2017), hindering a comparison with our record. The argument of Arienzo et al. (2017) that the biomass burning record in Antarctica primarily reflects changes in biomass burning rather than in atmospheric transport is consistent with the interpretation of the levoglucosan record in Talos Dome (see point (ii)).
3.5 Possible anthropogenic influence?
This Talos Dome levoglucosan record is limited by the available ice core samples and does not extend to the present, thereby not covering the time period of major regional population shifts, yet covering the time period of the advent of agriculture on a global scale. Several studies examine the anthropogenic influence on global to regional fire activity due to agriculture in the mid- to late Holocene (e.g., Broeker and Stocker, 2006; Joos et al., 2004; Singarayer et al., 2011; Mitchell et al., 2013; Kaplan et al., 2009, 2011). Although the first agricultural practices in South America likely appeared at ∼ 10 000 BP, the development of agriculture in the Southern Hemisphere was limited with respect to the Northern Hemisphere (Martin and Sauerborn, 2013). The HYDE 3.2 database demonstrates that in the possible fire source regions of Patagonia and southern Africa the demographic density was between 1 and 5 inhabitants km−2 and even less than 1 inhabitants km−2 in Australia until ∼ 1000 BP (Goldewijk, 2017). Human populations in the region fluctuated wildly from 1000 BP to present due to European settlement and the associated decimation of indigenous populations. The human impact on fire activity is not directly proportional to population density because small bands of humans can substantially change their environment, such as in the case of rapid burning of New Zealand forests (McWethy et al., 2010, 2014). However, the Talos Dome ice core record from 6000 to ∼ 750 BP currently does not provide clear evidence that the fire record may be strongly affected by anthropogenic activities during the mid- to late Holocene, although we cannot exclude at least a partial influence.
We determined the specific biomass burning biomarker levoglucosan in an ice core from TALDICE during the mid- to late Holocene (6000–750 BP). The levoglucosan record is characterized by a long-term increase with higher rates starting at ∼ 4000 BP and higher peaks between 2500 and 1500 BP. These peaks are consistent with other biomass burning records from Antarctica (Arienzo et al., 2017). Comparisons with regional charcoal syntheses suggested a primary contribution from southern South American fires rather than Australian biomass burning.
The location of Talos Dome provides an ideal situation to compare the biomass burning records of this ice core with ice cores from the interior of the continent as well as on the Antarctic Peninsula. Air masses reaching Antarctica are influenced by ENSO and SAM, and the relative importance of these atmospheric phenomena change through time and space, and therefore may influence the distribution of fire aerosols reaching each ice core location. Talos Dome is one end-member of the Ross Sea dipole (Bertler et al., 2018) for which during the last 1000 years, the stable isotopes of precipitation in TALDICE are in sync with SAMA. If this connection between SAMA and TALDICE stable isotopes remains consistent throughout the mid- to late Holocene, then our results demonstrate that biomass burning aerosols reaching TALDICE do not depend on the most prominent atmospheric phenomenon affecting the site. This coastal location and documented dipole (Bertler et al., 2018) suggests that the air masses affecting TALDICE are not always the same as those affecting internal or peninsular Antarctic sites. Changes in biomass burning, rather than changes in atmospheric transport are the primary influence on fire aerosols recorded in TALDICE. We determine that a generalized cooling period in the Southern Hemisphere at ∼ 2000 BP coincides with a shift to drier conditions in South America, characterized by initial conditions with available fuel, that, in turn, created favorable conditions for fire. Finally, although we cannot exclude a possible anthropogenic influence on the SH fire history, we did not find clear evidence for supporting this occurrence.
The dataset is available in the Supplement. Actually the .xls file includes the dataset reported in this paper.
The supplement related to this article is available online at: https://doi.org/10.5194/cp-14-871-2018-supplement.
The authors declare that they have no conflict of interest.
This work was financially supported by the Early Human Impact ERC Advanced
Grant of the European Commission's Seventh Framework Programme, grant number
267696, by Programma Nazionale di Ricerche in Antartide (PNRA) grant PEA
2013/B2.05, and by European Union Marie Curie IIF Fellowship
(MIF1-CT-2006-039529, TDICOSO) within the Seventh Framework Programme. The TALos
Dome Ice CorE (TALDICE) project, a joint European program, is funded by
national contributions from Italy, France, Germany, Switzerland and the
United Kingdom. Primary logistical support was provided by PNRA at Talos
Dome. The authors gratefully acknowledge the help of Elga LabWater in
providing the PURELAB Pulse and PURELAB Flex that produced the ultrapure
water used in these experiments. The authors thank Barbara Delmonte for
providing raw Talos Dome dust data. Any use of trade, firm or product names
is for descriptive purposes only and does not imply endorsement by the US
Government.
Edited by: Eric Wolff
Reviewed by: two anonymous referees
Abram, N. J., Mulvaney, R., Vimeux, F., Phipps, S. J., Turner, J., and England, M. H.: Evolution of the Southern Annular Mode during the past millennium, Nat. Clim. Change, 4, 564–569, 2014.
Akagi, S. K., Yokelson, R. J., Wiedinmyer, C., Alvarado, M. J., Reid, J. S., Karl, T., Crounse, J. D., and Wennberg, P. O.: Emission factors for open and domestic biomass burning for use in atmospheric models, Atmos. Chem. Phys., 11, 4039–4072, https://doi.org/10.5194/acp-11-4039-2011, 2011.
Albani, S., Delmonte, B., Maggi, V., Baroni, C., Petit, J.-R., Stenni B., Mazzola, C., and Frezzotti, M.: Interpreting last glacial to Holocene dust changes at Talos Dome (East Antarctica): implications for atmospheric variations from regional to hemispheric scales, Clim. Past, 8, 741–750, https://doi.org/10.5194/cp-8-741-2012, 2012a.
Albani, S., Mahowald, N. M., Delmonte, B., Maggi V., and Winckler G.: Comparing modeled and observed changes in mineral dust transport and deposition to Antarctica between the Last Glacial Maximum and current climates, Clim. Dynam., 38, 1731–1755, https://doi.org/10.1007/s00382-011-1139-5, 2012b.
Andreae, M. O. and Merlet, P.: Emission of trace gases and aerosols from biomass burning, Global Biogeochem. Cy., 15, 955–966, 2001.
Antony, R., Grannas, A. M., Willoughby, A. S., Sleighter, R. L., Thamban, M., and Hatcher, P. G.: Origin and Sources of Dissolved Organic Matter in Snow on the EastAntarctic Ice Sheet, Environ. Sci. Technol., 48, 6151–6159, 2014.
Archibald, S., Roy, D. P., van Wilgen, B., and Scholes, R. J.: What limits fire? An examination of drivers of burnt area in Southern Africa, Glob. Change Biol., 15, 613–630, 2009.
Arienzo, M. M., McConnell, J. R., Murphy, L. N., Chellman, N., Das, S., Kipfstuhl, S., and Mulvaney, R.: Holocene black carbon in Antarctica paralleled Southern Hemisphere climate, J. Geophys. Res.-Atmos., 122, 6713–6728, https://doi.org/10.1002/2017JD026599, 2017.
Bai, J., Sun, X., Zhang, C., Xu, Y., and Qi, C.: The OH-initiated atmospheric reaction mechanism and kinetics for levoglucosan emitted in biomass burning, Chemosphere, 93, 2004–2010, https://doi.org/10.1016/j.chemosphere.2013.07.021, 2013.
Bakan, S., Chlond, A., Cubasch, U., Feichter, J., Graf, H., Grassl, H., Hasselmann, K., Kirchner, I., Latif, M., Roeckner, E., Sausen, R., Schlese, U., Schriever, D., Schumann, U., Sielmann, F., and Welke, W.: Climate response to smoke from the burning oil wells in Kuwait, Nature, 351, 367–371, 1991.
Basile, I., Grousset, F. E., Revel, M., Petit, J.-R., Biscaye, P. E., and Barkov, N. I.: Patagonian origin of glacial dust deposited in East Antarctica (Vostok and Dome C) during glacial stages 2, 4 and 6, Earth Planet. Sc. Lett., 146, 573–589, 1997.
Bauer, S. E., Bausch, A., Nazarenko, L., Tsigardis, K., Xu, B., Edwards, R., Bisiaux, M., and McConnell, J.: Historical and future black carbon deposition on the three ice caps: Ice core measurements and model simulations from 1850 to 2100, J. Geosphys. Res.-Atmos., 118, 7948–7961, https://doi.org/10.1002/jgrd.50612, 2013
Bazin, L., Landais, A., Lemieux-Dudon, B., Toyé Mahamadou Kele, H., Veres, D., Parrenin, F., Martinerie, P., Ritz, C., Capron, E., Lipenkov, V., Loutre, M.-F., Raynaud, D., Vinther, B., Svensson, A., Rasmussen, S. O., Severi, M., Blunier, T., Leuenberger, M., Fischer, H., Masson-Delmotte, V., Chappellaz, J., and Wolff, E.: An optimized multi-proxy, multi-site Antarctic ice and gas orbital chronology (AICC2012): 120–800 ka, Clim. Past, 9, 1715–1731, https://doi.org/10.5194/cp-9-1715-2013, 2013.
Bertler, N. A. N., Conway, H., Dahl-Jensen, D., Emanuelsson, D. B., Winstrup, M., Vallelonga, P. T., Lee, J. E., Brook, E. J., Severinghaus, J. P., Fudge, T. J., Keller, E. D., Baisden, W. T., Hindmarsh, R. C. A., Neff, P. D., Blunier, T., Edwards, R., Mayewski, P. A., Kipfstuhl, S., Buizert, C., Canessa, S., Dadic, R., Kjaer, H. A., Kurbatov, A., Zhang, D., Waddington, E. D., Baccolo, G., Beers, T., Brightley, H. J., Carter, L., Clemens-Sewall, D., Ciobanu, V. G., Delmonte, B., Eling, L., Ellis, A., Ganesh, S., Golledge, N. R., Haines, S., Handley, M., Hawley, R. L., Hogan, C. M., Johnson, K. M., Korotkikh, E., Lowry, D. P., Mandeno, D., McCay, R. M., Menking, J. A., Naish, T. R., Noerling, C., Ollive, A., Orsi, A., Proemse, B. C., Pyne, A. R., Pyne, R. L., Renwick, J., Scherer, R. P., Semper, S., Simonsen, M., Sneed, S. B., Steig, E. J., Tuohy, A., Venugopal, A. U., Valero-Delgado, F., Venkatesh, J., Wang, F., Wang, S., Winski, D. A., Winton, H. L., Whiteford, A., Xiao, C., Yang, J., and Zhang, X.: The Ross Sea Dipole – temperature, snow accumulation and sea ice variability in the Ross Sea region, Antarctica, over the past 2700 years, Clim. Past., 14, 193–214, https://doi.org/10.5194/cp-14-193-2018, 2018.
Bisiaux, M. M., Edwards, R., McConnell, J. R., Curran, M. A. J., Van Ommen T. D., Smith, A. M., Neumann, T. A., Pasteris, D. R., Penner, J. E., and Taylor, K.: Changes in black carbon deposition to Antarctica from two high-resolution ice core records, 1850–2000 AD, Atmos. Chem. Phys., 12, 4107–4115, https://doi.org/10.5194/acp-12-4107-2012, 2012.
Blarquez, O., Vannière, B., Marlon, J. R., Daniau, A.-L., Power, M. J., Brewer, S., and Bartlein, P. J.: An R package to analyse sedimentary charcoal records from the Global Charcoal Database to reconstruct past biomass burning, Comput. Geosci., 72, 255–261, 2014.
Bowman, D. M. J., Balch, J. K., Artaxo, P., Bond, W. J., Carlson, J. M., Cochrane, M. A., D'Antonio, C. M., DeFries, R. S., Doyle, J. C., Harrison, S. P., Johnston, F. H., Keeley, J. E., Krawchuk, M. A., Kull, C. A., Marston, J. B., Moritz, M. A., Prentice, I. C., Roos, C. I., Scott, A. C., Swetnam, T. W., van der Werf, G. R., and Pyne, S. J.: Fire in the Earth system, Science, 324, 481–484, 2009.
Broecker, W. S. and Stocker, T. F.: The Holocene CO2 rise: Anthropogenic or natural?, Eos Trans. AGU, 87, 27–27, https://doi.org/10.1029/2006EO030002, 2006.
Brunelle, A. and Whitlock, C.: Postglacial fire, vegetation, and climate history in the Clearwater Range, Northern Idaho, USA, Quaternary Res., 60, 307–318, 2003.
Buiron, D., Chappellaz, J., Stenni, B., Frezzotti, M., Baumgartner,M., Capron, E., Landais, A., Lemieux-Dudon, B., Masson-Delmotte, V., Montagnat, M., Parrenin, F., and Schilt, A.: TALDICE-1 age scale of the Talos Dome deep ice core, East Antarctica, Clim. Past, 7, 1–16, https://doi.org/10.5194/cp-7-1-2011, 2011
Canty, A. and Ripley, B.: Bootstrap R (S-Plus) Functions, R package version 1.3-20, 2017.
Carcaillet, C., Bouvier, M., Fréchette, B., Larouche, A. C., and Richard, P. J. H. : Comparison of pollen-slide andsieving methods in lacustrine charcoal analyses for local and regional fire history, The Holocene, 11, 467–476,2001.
Carcaillet, C., Almquist, H., Asnong, H., Bradshaw, R. H. W., Carrión, J. S., Gaillard, M. J., Gajewski, K., Haas, J. N., Haberle, S. G., Hadorn, P., Müller, S. D., Richard, P. J. H., Richoz, I., Rösch, M., Sánchez Goñi, M. F., Von Stedingk, H., Stevenson, A. C., Talon, B., Tardy, C., Tinner, W., Tryterud, E., Wick, L., and Willis, K. J. : Holocene biomass burning and global dynamics of the carbon cycle, Chemosphere, 49, 845–863, 2002.
Christie, D. A., Boninsegna, J. A., Cleaveland, M. K., Laral, A., Le Quesne, C., Morales, M. A., Mudelsee, M., Stahle, D. W., and Villalba, R.: Aridity changes in the temperate-Mediterranean transition of the Andes since AD 1346 reconstructed from tree-rings, Clim. Dynam., 36, 1505–1521, 2011.
Chuvieco, E., Giglio, L., and Justice, C.: Global characterization of fire activity: toward defining fire regimes from Earth observation data, Glob. Change Biol. 14, 1488–1502, 2008.
Clare-Smith, M., Singarayer, J. S., Valdes, P. J., Kaplan, J. O., and Branch, N. P.: The biogeophysical climatic impacts of anthropogenic land use change during the Holocene, Clim. Past, 12, 923–941, https://doi.org/10.5194/cp-12-923-2016, 2016.
Clark, J. S.: Particle motion and the theory of charcoal analysis: source area, transport, deposition, and sampling, Quaternary Res., 30, 67–80, 1988.
Clark, J. S., Stocks, B. J., and Richard, P. J. H.: Climate implications of biomass burning since the 19th century in eastern North America, Glob. Change Biol., 2, 433–442, 1996.
Cook, R. D.: Detection of Influential Observations in Linear Regression, Technometrics, 19, 15–18, 1977.
Correia, A., Freydier, R., Delmas, R. J., Simões, J. C., Taupin, J.-D., Dupré, B., and Artaxo, P.: Trace elements in South America aerosol during 20th century inferred from a Nevado Illimani ice core, Eastern Bolivian Andes (6350 m a.s.l.), Atmos. Chem. Phys., 3, 1337–1352, https://doi.org/10.5194/acp-3-1337-2003, 2003.
Daniau, A.-L., Harrison, S. P., and Bartlein, P. J.: Fire regimes during the Last Glacial, Quateranry Sci. Rev., 29, 2918–2930, 2010.
Daniau, A.-L., Bartlein, P. J., Harrison, S. P., Prentice, I. C., Brewer, S., Friedlingstein, P., Harrison-Prentice, T. I., Inoue, J., Izumi, K., Marlon, J. R., Mooney, S., Power, M. J., Stevenson, J.,Tinner, W., Andri, M., Atanassova, J., Behling, H., Black, M., Blarquez, O., Brown, K. J., Carcaillet, C., Colhoun, E. A., Colombaroli, D., Davis, B. A. S., D'Costa, D., Dodson, J., Dupont, L., Eshetu, Z., Gavin, D .G., Genries, A., Haberle, S., Hallett, D. J., Hope, G., Horn, S. P., Kassa, T. G., Katamura, F., Kennedy, L. M., Kershaw, P., Krivonogov, S., Long, C.,Magri, D., Marinova, E., McKenzie, G. M., Moreno, P. I., Moss, P., Neumann, F. H., Norström, E., Paitre, C., Rius, D., Roberts, N., Robinson, G. S., Sasaki, N., Scott, L., Takahara, H.,Terwilliger, V., Thevenon, F., Turner, R., Valsecchi, V. G., Vannière, B., Walsh, M., Williams, N., and Zhang, Y.: Predictability of biomass burning in response to climate changes, Global Biogeochem. Cy., 26, GB4007, https://doi.org/10.1029/2011GB004249, 2012.
Davison, A. C. and Hinkley, D. V.: Bootstrap Methods and Their Applications. Cambridge University Press, Cambridge, ISBN 0-521-57391-2, 1997.
de Montford, M.: Late Holocene fire history: Human impacts and climate variability at Wingecarribee Swamp, Unpublished BSc Hons Thesis, School of Biological, Earth & Environmental Sciences, UNSW, Sydney, Reference in GCD, available at: http://www.paleofire.org (last access: 11 July 2017), 2008.
Delmonte, B., Petit, J.-R., Krinner, G., Maggi, V., Jouzel, J., and Udisti, R.: Ice core evidence for secular variability and 200-year dipolar oscillations in atmospheric circulation over East Antarctica during the Holocene, Clim. Dynam., 24, 641–654, 2005.
Delmonte, B., Petit, J. R., Basile-Doelsch, I., Jagoutz, E., and Maggi, V.: Late quaternary interglacials in East Antarctica from ice-core dust records, Dev. Quat. Sci., 7, 53–73, https://doi.org/10.1016/S1571-0866(07)80031-5, 2007.
Delmonte, B., Delmas, R. J., and Petit, J.-R.: Comment on “Dust provenance in Antarctic ice during glacial periods: from where in southern South America?” by D. M. Gaiero, Geophys. Res. Lett., 35, L08707, https://doi.org/10.1029/2007GL032075, 2008.
Delmonte, B., Andersson, P. S., Schoberg, H., Hansson, M., Petit, J.-R., Delmas, R., Gaiero, D. M., Maggi, V., and Frezzotti, M.: Geographic provenance of aeolian dust in East Antarctica during Pleistocene glaciations: preliminary results from Talos Dome and comparison with East Antarctic and new Andean ice core data, Quateranry Sci. Rev., 29, 256–264, 2010a.
Delmonte, B., Baroni, C., Andersson, P.S., Schoberg, H., Hansson, M., Aciego, S., Petit, J.-R., Albani, S., Mazzola, C., Maggi, V., and Frezzotti, M.: Aeolian dust in the Talos Dome ice core (East Antarctica, Pacific/Ross Sea sector): Victoria Land versus remote sources over the last two climate cycles, J. Quateranry Sci., 25, 1327–1337, https://doi.org/10.1002/jqs.1418, 2010b.
Delmonte, B., Baroni, C., Andersson, P. S., Narcisi, B., Salvatore, M. C., Petit, J. R., Scarchilli, C., Frezzotti, M., Albani, S., and Maggi, V.: Modern and Holocene aeolian dust variability from Talos Dome (Northern Victoria Land) to the interior of the Antarctic ice sheet, Quaternary Sci. Rev., 64, 76e89, https://doi.org/10.1016/j.quascirev.2012.11.033, 2013.
Fiebig, M., Lunder, C. R., and Stohl, A.: Tracing biomass burning aerosol from South America to Troll Research Station, Antarctica, Geophys. Res. Lett., 36, L14815, https://doi.org/10.1029/2009GL038531, 2009.
Fine, P. M., Cass, G. R., and Simoneit, B. R. T.: Chemical characterization of the fine particle emissions from fireplacecombustion of woods grown in the southern United States, Environ. Sci. Technol., 36, 1442–1451, 2002.
Flanner, M. G., Zender, C. S., Randerson, J. T., and Rasch, P. J.: Present-day climate forcing and response from black carbon in snow, J. Geophys. Res., 112, D11202, https://doi.org/10.1029/2006JD008003, 2007.
Gabrielli, P., Wegner, A., Petit, J.-R., Delmonte, B., DeDeckker, P., Gaspari, V., Fisher, H., Ruth, U., Kriews, M., Boutron, C., Cescon, P., and Barbante, C.: A major glacial-interglacial change in aeolian dust composition inferred from Rare Earth Elements in Antarctica ice, Quaternary Sci. Rev., 29, 265–273, https://doi.org/10.1016/j.quascirev.2009.09.002, 2010.
Gaiero, D. M.: Dust provenance in Antarctic ice during glacial periods: From where in southern South America?, Geophys. Res. Lett., 34, L17707, https://doi.org/10.1029/2007GL030520, 2007.
Galanter, M., Levy, H., and Carmichael, G. R.:Impacts of biomass burning on tropospheric CO, NOX, and O3, J. Geophys. Res., 105, 6633–6653, https://doi.org/10.1029/1999JD901113, 2000.
Gambaro, A., Zangrando, R., Gabrielli, P., Barbante, C., and Cescon, P.: Direct Determination of Levoglucosan at the Picogram per Milliliter Level in Antarctic Ice by High-Performance Liquid Chromatography/Electrospray Ionization Triple Quadrupole Mass Spectrometry, Anal. Chem., 80, 1649–1655, 2008.
Goldewijk, K.: Anthropogenic land-use estimates for the Holocene; HYDE 3.2. DANS, https://doi.org/10.17026/dans-25g-gez3, 2017.
Grousset, F. E., Biscaye, P. E., Revel, M., Petit, J.-R., Pye, K., Joussaume, S., and Jouzel, J.: Antarctic (Dome C) ice-core dust at 18 k.y.B.P.: Isotopic constraints on origins, Earth Planet. Sc. Lett., 111, 175–182, 1992.
Hall, B. L.: Holocene glacial history of Antarctica and the sub-Antarctic islands, Quateranry Sci. Rev., 28, 2213–2230, 2009.
Hara, K., Osada, K., Yabuki, M., Hashida, G., Yamanouchi, T., Hayashi, M., Shiobara, M., Nishita, C., and Wada, M.: Haze episodes at Syowa Station, coastal Antarctica: Where did they come from?, J. Geophys. Res., 115, D14205, https://doi.org/10.1029/2009JD012582, 2010.
Harrison, S. P., Marlon, J. R., and Bartlein, P. J.: Fire in the Earth system, in: Changing Climates, edited by: Dodson, J., Earth Systems and Society, Springer, Dordrecht, the Netherlands, 21–48, https://doi.org/10.1007/978-90-481-8716-4_3, 2010.
Hennigan, C. J., Sullivan, A. P., Collett, J. L., and Robinson, A. L.: Levoglucosan stability in biomass burning particles exposed to hydroxyl radicals, Geophys. Res. Lett., 37, L09806, https://doi.org/10.1029/2010gl043088, 2010.
Hoffmann, D., Tilgner, A., Iinuma, Y., and Herrmann, H.: Atmospheric Stability of Levoglucosan: A Detailed Laboratory and Modeling Study, Environ. Sci. Technol., 44, 694–699, https://doi.org/10.1021/es902476f, 2010.
Hu, Q.-H., Xie, Z.-Q., Wang, X.-M., Kang, H., He, Q.-F., and Zhang, P.: Secondary organic aerosols over oceans via oxidation of isoprene and monoterpenes from Arctic to Antarctic, Sci. Rep., 3, 2280, https://doi.org/10.1038/srep02280, 2013.
Iglesias, V. and Whitlock, C.: Fire responses to postglacial climate change and human impact in northern Patagonia (41–43∘ S), P. Natl. Acad. Sci. USA, 8, E5545–E5554, 2014.
Iglesias, V., Whitlock, C., Markgraf, V., and Bianchi, M. M.: Postglacial history of the Patagonian forest/steppe ecotone (41–43∘ S), Quateranry Sci. Rev., 94, 120–135, 2014.
Indermühle, A., Stocker, T. F., Joos, F., Fischer, H., Smith, H. J., Wahlen, M., Deck, B., Mastroianni, D., Tschumi, J., Blunier, T., Meyer, R., and Stauffer, B.: Holocene carbon-cycle dynamics based on CO2 trapped in ice at Taylor Dome, Antarctica, Nature, 398, 121–126, 1999.
Joos, F., Gerber, S., Prentice, I. C., Otto-Bliesner, B. L., and Valdes, P. J.: Transient simulations of Holocene atmosphericcarbon dioxide and terrestrial carbon since the lastglacial maximum, Global Biogeochem. Cy., 18, GB2002, https://doi.org/10.1029/2003GB002156, 2004.
Kaplan, J. O., Krumhardt, K. M., and Zimmermann, N.: The prehistoric and preindustrial deforestation of Europe, Quaternary Sci. Rev., 28, 3016–3034, 2009.
Kaplan, J. O., Krumhardt, K. M., Ellis, E. C., Ruddiman, W. F., Lemmen, C., and Goldewijk, K. K.: Holocene carbon emissions as a result ofanthropogenic land cover change, The Holocene, 21, 775–791, 2011.
Kawamura, K., Narukawa, M., Li, S.-M., and Barrie, L. A.: Size distributions of dicarboxylic acids and inorganic ions inatmospheric aerosols collected during polar sunrise in the Canadian high Arctic, J. Geophys. Res., 112, D10307, https://doi.org/10.1029/2006JD008244, 2007.
Kehrwald, N., Zangrando, R., Gabrielli, P., Jaffrezo, J. L., Boutron, C., Barbante, C., and Gambaro, A.: Levoglucosan as a specific marker of fire events in Greenland snow, Tellus B, 64, 18196, https://doi.org/10.3402/tellusb.v64i0.18196, 2012.
Keywood, M., Kanakidou, M., Stohl, A., Dentener, F., Grassi, G., and Meyer, C. P.: Fire in the air: Biomass burning impacts in a changing climate, Crit. Rev. Environ. Sci. Technol., 43, 40–83, https://doi.org/10.1080/10643389.2011.604248, 2013.
Kuo, J.-L., Herbert, B. E., and Louchouarn, P.: Can levoglucosan be used to characterize and quantify char/charcoal black carbon in environmental media?, Org. Geochem, 39, 1466–1478, 2008.
Lambert, F., Delmonte, B., Petit, J.-R., Bigler, M., Kaufmann, P. R., Hutterli, M. A., Stocker, T. F., Ruth, U., Steffensen, J. P., and Maggi, V.: Dustclimate couplings over the past 800,000 years from the EPICA Dome C ice core, Nature, 452, 616–619, https://doi.org/10.1038/nature06763, 2008.
Lamy, F., Hebbeln, D., Rohl, U., and Wefer, G.: Holocene rainfall variability in southern Chile: A marine record of latitudinal shifts of the southern westerlies, Earth Planet. Sc. Lett., 185, 369–382, 2001.
Lamy, F., Gersonde, R., Winkler, G., Esper, O., Jaeschke, A., Kuhn, G., Ullermann, J., Martinez-Garcia, A., Lambert, F., and Kilian, R.: Increased Dust Deposition in the Pacific Southern Ocean During Glacial Periods, Science, 343, 403–407, https://doi.org/10.1126/science.1245424, 2014.
Laskar, J. P., Robutel, P., Joutel, F., Gastrineau, M., Correia, A., and Levrard, B.: A long term numerical solution for the insolation quantities of the Earth, Astron. Atrophys., 428, 261–285, 2004.
Lee, Y. H., Lemarque, J.-F., Flanner, M. G., Jiao, C., Shindell, D. T., Berntsen, T., Bisiaux, M. M., Cao, J., Collins, W. J., Curran, M., Edwards, R., FAluvegi, G., Ghan, S., Horowitz, L. W., McConnell, J. R., Ming, J., Myhre, G., Nagashima, T., Naik, V., Rumbold, S. T., Skeie, R. B., Sudo, K., Takemura, T., Thevenon, F., Xu, B., and Yoon, J.-H.: Evaluation of preindustrial to present-day black carbon and its albedo forcing from ACCMIP (Atmospheric Chemistry and Climate Model Intercomparison Project), Atmos. Chem. Phys., 12, 21713–21778, https://doi.org/10.5194/acp-13-2607-2013, 2013.
Legrand, M., De Angelis, M., Staffelbach, T., Neftel, A., and Stauffer, B.: Large perturbation of ammonium and organic-acids content in the Summit-Greenland ice core: Fingerprint from forest fires, Geophys. Res. Lett., 19, 473–475, 1992.
Legrand, M., McConnell, J., Fischer, H., Wolff, E. W., Preunkert, S., Arienzo, M., Chellman, N., Leuenberger, D., Maselli, O., Place, P., Sigl, M., Schüpbach, S., and Flannigan, M.: Boreal fire records in Northern Hemisphere ice cores: a review, Clim. Past, 12, 2033–2059, https://doi.org/10.5194/cp-12-2033-2016, 2016.
Lynch, J. A., Hollis, J. L., and Hu, F. S.: Climatic and landscape controls of the boreal forest fire regime: Holocene records from Alaska, J. Ecol., 92, 477–189, 2004.
Marcott, S. A., Shakun, J. D., Clark, P. U., and Mix, A. C.: A reconstruction of regional and global temperature for the past 11,300 Years, Science, 339, 1198–1201, 2013.
Marlon, J. R., Bartlein, J., Carcaillet, C., Gavin, D., Harrison, S., Higuera, P., Joos, F., Power, M., and Prentice, I. C.: Climate and human influences on global biomass burning over the past two millennia, Nat. Geosci. 1, 697–702, 2008.
Marlon, J. R., Bartlein, P. J., Daniau, A. L., Harrison, S. P., Maezumi, S. Y., Power, M. J., Tinner, W., and Vanniere, B.: Global biomass burning: a synthesis and review of Holocene paleofire records and their controls, Quaternary Sci. Rev., 65, 5–25, https://doi.org/10.1016/j.quascirev.2012.11.029, 2013.
Marlon, J. R., Kelly, R., Daniau, A.-L., Vannière, B., Power, M. J., , Bartlein, P., Higuera, P., Blarquez, O., Brewer, S., Brücher, T., Feurdean, A., Romera, G. G., Iglesias, V., Maezumi, S. Y., Magi, B., Mustaphi, C. J. C., and Zhihai, T.: Reconstructions of biomass burning from sediment-charcoal records to improve data–model comparisons, Biogeosciences, 13, 3225–3244, https://doi.org/10.5194/bg-13-3225-2016, 2016.
Marshall, G. J.: Trends in the Southern Annular Mode from observations and reanalyses, J. Clim., 16, 4134–4143, 2003.
Marshall, G. J. and Thompson, D. W. J.: The signatures of large-scale patterns of atmospheric variability in Antarctic surface temperatures, J. Geophys. Res.-Atmos., 121, 3276–3289, https://doi.org/10.1002/2015jd024665, 2016.
Masson-Delmotte, V., Buiron, D., Ekaykin, A., Frezzotti, M., Gallẽe, H., Jouzel, J., Krinner, G., Landais, A., Motoyama, H., Oerter, H., Pol, K., Pollard, D., Ritz, C., Schlosser, E., Sime, L. C., Sodemann, H., Stenni, B., Uemura, R., and Vimeux, F.: A comparison of the present and last interglacial periods in six Antarctic ice cores, Clim. Past, 7, 397–423, https://doi.org/10.5194/cp-7- 397-2011, 2011.
Martin, K. and Sauerborn, J.: Chapter 2 in Agroecology: Origin and development of agriculture, Springer Science/Business Media B.V., Dordrecht, 2013.
Mayewski, P. A., Carleton, A. M., Birkel, S. D., Dixon, D., Kurbatov, A. V., Korotkikh, E., McConnell, J., Curran, M., Cole-Dai, J., Jiang, S., Plummer, C., Vance, T., Maasch, K. A., Sneed, S. B., and Handley, M.: Ice core and climate reanalysis analogs to predict Antarctic and Southern Hemisphere climate changes, Quaternary Sci. Rev., 155, 50–56, https://doi.org/10.1016/j.quascirev.2016.11.017, 2017.
McConnell, J. R., Edwards, R., Kok, G. L., Flanner, M. G., Zender, C. S., Saltzman, E. S., Banta, J. R., Pasteris,D. R., Carter, M. M., and Kahl, J. D. W.: 20th-century industrial black carbon emissions altered arctic climateforcing, Science, 317, 1381–1384, https://doi.org/10.1126/science.1144856, 2007.
McWethy, D. B., Wilmshurst, J. M., Whitlock, C., Wood, J. R., and McGlone, M. S.: A high-resolution chronology of rapid forest transitions following polynesian arrival in New Zealand, PLoS One, 5, e111328, https://doi.org/10.1371/journal.pone.0111328, 2014.
McWethy, D. B., Whitlock, C., Wilmshurst, J. M., McGlone, M. S., Fromont, M., Li, X., Dieffenbacher-Krall, A., Hobbse, W. O., Fritz, S. C., and Cook, E. R.: Rapid landscape transformation in South Island, New Zealand, following initial Polynesian settlement, P. Natl. Acad. Sci. USA, 107, 21343–21348, https://doi.org/10.1073/pnas.1011801107, 2010.
Mitchell, L., Brook, E., Lee, J., Buizert, C., and Sowers, T.: Constraints on the late Holocene anthropogenic contributionto the atmospheric methane budget, Science, 342, 964–966, https://doi.org/10.1126/science.1238920, 2013.
Mooney, S., Harrison, S., Bartlein, P., Daniau, A.-L., Stevenson, J., Brownlie, K., Buckman, S., Cupper, M., Luly, J., and Black, M.: Late Quaternary fire regimes of Australasia, Quaternary Sci. Rev., 30, 28–46, 2011.
Moy, C. M., Seltzer, G. O., Rodbell, D. T., and Anderson, D. M.: Variability of El Niño/Southern Oscillation activity at millennial timescales during the Holocene epoch, Nature, 420, 162–165, 2002.
Mundo, I. A., Villalba, R., Veblen, T. T., Kitzberger, T., Holz, A., Paritsis, J., and Ripalta, A.: Fire history in southern Patagonia: human and climate influences on fire activity in Nothofagus pumilo forests, Ecosphere, 8, e01932, https://doi.org/10.1002/ecs2.1932, 2017.
Ogden, J., Baher, L., and McGlone, M.: Fire, forest regeneration and links with Early Human habitation: evidence from New Zealand, Ann. Bot., 81, 687–696, 1998.
Pasteris, D. R., McConnell, J. R., Das, S. B., Criscitiello, A. S., Evans, M. J., Maselli, Sigl, M., and Layman, L.: Seasonally resolved ice core records from West Antarctica indicate a sea ice source of sea-salt aerosol and a biomass burning source of ammonium, J. Geophys. Res.-Atmos., 119, 9168–9182, https://doi.org/10.1002/2013JD020720, 2014.
Petit, J.-R., Jouzel, J., Raynaud, D., Barkov, N. I., Barnola, J. M., Basile, I., Bender, M., Chappellaz, J., Davisk, M., Delaygue, G., Delmotte, M., Kotlyakov, V. M., Legrand, M., Lipenkov, V. Y., Lorius, C., Pepin, L., Ritz, C., Saltzmank, E., and Stievenard, M.: Climate and atmospheric history of the past 420,000 years from the Vostok ice core, Antarctica, Nature, 399, 429–436, 1999.
Pickett, E., Harrison, S. P., Hope, G., Harle, K., Dodson, J. R., Kershaw, A. P., Prentice, I. C., Backhouse, J., Colhoun, E. A., D'Costa, D., Flenley, J., Grindrod, J., Haberle, S., Hassell, C., Kenyon, C., Macphail, M., Martin, H., Martin, A. H., McKenzie, M., Newsome, J. C., Penny, D., Powell, J., Raine, I., Southern, W., Stevenson, J., Sutra, J. P., Thomas, I., van der Kaars, S., Walker, D., and Ward, J.: Pollen-based reconstructions of biome distributions for Australia, south east Asia and the Pacific (SEAPAC region) at 0, 6000 and 18,000 14C yr B.P., J. Biogeogr., 31, 1381–1444, https://doi.org/10.1111/j.1365-2699.2004.01001.x, 2004.
Power, M., Marlon, J., Ortiz, N., Bartlein, P., Harrison, S., Mayle, F., Ballouche, A., Bradshaw, R., Carcaillet, C., Cordova, C., Mooney, S., Moreno, P., Prentice, I., Thonicke, K., Tinner,W., Whitlock, C., Zhang, Y., Zhao, Y., Ali, A., Anderson, R., Beer, R., Behling, H., Briles, C., Brown, K., Brunelle, A., Bush, M., Camill, P., Chu, G., Clark, J., Colombaroli, D., Connor, S., Daniau, A. L., Daniels, M., Dodson, J., Doughty, E., Edwards, M., Finsinger, W., Foster, D., Frechette, J., Gaillard, M. J., Gavin, D., Gobet, E., Haberle, S., Hallett, D.,Higuera, P., Hope, G., Horn, S., Inoue, J., Kaltenrieder, P., Kennedy, L., Kong, Z., Larsen, C., Long, C., Lynch, J., Lynch, E., McGlone, M., Meeks, S., Mensing, S., Meyer, G., Minckley, T., Mohr, J., Nelson, D., New, J., Newnham, R., Noti, R., Oswald,W., Pierce, J., Richard, P., Rowe, C., Sanchez Goñi, M., Shuman, B., Takahara, H., Toney, J., Turney, C., Urrego-Sanchez, D., Umbanhowar, C., Vandergoes, M., Vanniere, B., Vescovi, E., Walsh, M., Wang, X., Williams, N., Wilmshurst, J., and Zhang, J.: Changes in fire regimessince the Last Glacial Maximum: an assessment based on a global synthesis and analysis of charcoal data, Clim. Dynam., 30, 887–907,2008.
Power, M., Marlon, J., Bartlein, P., and Harrison, S.: Fire history and the Global Charcoal Database: a new tool for hypothesis testing and data exploration, Palaeogeogr. Palaeocl., 291, 52–59, 2010.
Power, M., Mayle, F., Bartlein, P., Marlon, J., Anderson, R., Behling, H., Brown, K., Carcaillet, C., Colombaroli, D., and Gavin, D.: Climatic control of the biomass-burning declinein the Americas after AD 1500, The Holocene, 23, 3–13, 2013.
Pyne, S. J.: The fires this time, and next, Science, 294, 1005–1006, 2001.
R Core Team: R: A language and environment for statistical computing. R Foundation for Statistical Computing, Vienna, Austria, https://www.R-project.org/, last access: 12 November 2017.
Rehfeld, K., Marwan, N., Heitzig, J., and Kurths, J.: Comparison of correlation analysis techniques for irregularly sampled time series, Nonlinear Proc. Geoph., 18, 389–404, 2011.
Revel-Rolland, M., De Deckker, P., Delmonte, P., Hesse, P. P., Magee, J. W., Basile-Doelsch, I., Grousset, F., and Bosch, D.: Eastern Australia: a possible source of dust in East Antarctica interglacial ice, Earth Planet Sc. Lett., 249, 1–13, 2006.
Roberts, J., Plummer, C., Vance, T., Van Ommen, T., Moy, A., Poynter, S., Treverrow, A., Curran, M., and George, S.: A 2000-year annual record of snow accumulation rates for Law Dome, East Antarctica, Clim. Past, 11, 697–707, doi.org/10.5194/cp-11-697-2015
Rollins, M. G., Morgan, P., and Swetnam, T.: Landscape scale controls over 20th century fire occurrence in two large Rocky Mountain (USA) wilderness areas, Landscape Ecol., 17, 539–557, 2002.
Rubino, M., D'Onofrio, A., Seki, O., and Bendle, J. A.: Ice-core records of biomass burning, The Anthropocene Review, 3, 140–162, https://doi.org/10.1177/2053019615605117, 2016.
Ruddiman, W. F.: The anthropogenic greenhouse era began thousands of years ago, Clim. Change, 61, 261–293, https://doi.org/10.1023/B:CLIM.0000004577.17928.fa, 2003.
Schneider, D. P. and Steig, E. J.: Ice cores record significant 1940s Antarctic warmth related to tropical climate variability, P. Natl. Acad. Sci. USA, 105, 12154–12158, https://doi.org/10.1073/pnas.0803627105, 2008.
Siggaard-Andersen, M.-L., Gabrielli, P., Steffensen, J. P., Strømfeldt, T., Barbante, C., Boutron, C., Fischer, H., and Miller, H.: Soluble and insoluble lithium dust in the EPICA DomeC ice core: Implications for changes of the East Antarctic dust provenance during the recent glacial interglacial transition, Earth Planet. Sc. Lett., 258, 32–43, 2007.
Singarayer, J. S., Valdes, P. J., Friedlingstein, P., Nelson, S., and Beerling, D. J.: Late Holocene methane rise caused by orbitallycontrolled increase in tropical sources, Nature, 470, 82–85, https://doi.org/10.1038/nature09739, 2011.
Slade, J. H. and Knopf, D. A.: Heterogeneous OH oxidation of biomass burning organic aerosols surrogate compounds: assessment of volatilization products and the role of OH concentration on the reactive uptake kinetics, Phys. Chem. Chem. Phys., 15, 5898–5915, https://doi.org/10.1039/c3cp44695f, 2013.
Stenni, B., Proposito, M., Gragnani, R., Flora, O., Jouzel, J., Falourd, S., and Frezzotti, M.: Eight centuries of volcanic signal and climate change at Talos Dome (East Antarctica), J. Geophys Res., 107, D94076, https://doi.org/10.1029/2000JD000317, 2002.
Stenni, B., Buiron, D., Frezzotti, M., Albani, S., Barbante, C., Bard, E., Barnola, J. M., Baroni, M., Baumgartner, M., Bonazza, M., Capron, E., Castellano, E., Chappellaz, J., Delmonte, B., Falourd, S., Genoni, L., Iacumin, P., Jouzel, J., Kipfstuhl, S., Landais, A., Lemieux-Dudon, B., Maggi, V., Masson-Delmotte, V., Mazzola, C., Minster, B., Montagnat, M., Mulvaney, R., Narcisi, B., Oerter, H., Parrenin, F., Petit, J. R., Ritz, C., Scarchilli, C., Schilt, A., Schũpbach, S., Schwander, J., Selmo, E., Severi, M., Stocker, T. F., and Udisti, R.: Expression of the bipolar seesaw in Antarctic climate records during the last deglaciation, Nat. Geosci., 4, 46–49, https://doi.org/10.1038/ngeo1026, 2011.
Tauger, M. B.: Agriculture in world history (in Themes in world history), Chapter 1, 3–14, Milton Park, England, Routledge, 2011.
Vallelonga, P., Gabrielli, P., Balliana, E., Wegner, A., Delmonte, B., Buretta, C., Burton, G., Vanhaecke, F., Rosman, K. J. R., Hong, S., Boutron, C.F., Cescon, P., and Barbante, C.: Lead isotopic compositions in the EPICA Dome C ice core and SouthernHemisphere Potential Source Areas, Quaternary Sci. Rev., 29, 247–255, https://doi.org/10.1016/j.quascirev.2009.06.019, 2010.
van der Werf, G. R., Randerson, J. T., Collatz, G. J., Giglio, L., Kasibhatla, P. S., Arellano Jr., A. F., Olsen, S. C., and Kasischke, E. S.: Continental-scale partitioning of fireemissions during the 1997 to 2001 El Niño/La Niña period, Science, 303, 73–76, https://doi.org/10.1126/science.1090753, 2004.
Vannière, B., Power, M. J., Roberts, N., Tinner, W., Carrión, J., Magny, M., Bartlein, P., Colombaroli, D., Daniau, A., and Finsinger, W.: Circum-Mediterranean fire activity andclimate changes during the mid-Holocene environmental transition (8500–2500 cal. BP), The Holocene, 21, 53–73, 2011.
Veres, D., Bazin, L., Landais, A., Mahamadou Kele, H. T., Lemieux-Dudon, B., Parrenin, F., Martinerie, P., Blayo, E., Blunier, T., Capron, E., Chappellaz, J., Rasmussen, S. O., Severi, M., Svensson, A., Vinther, B., and Wolff, E. W.: The Antarctic ice core chronology (AICC2012): an optimized multi-parameter and multi-site dating approach for the last 120 thousand years, Clim. Past, 9, 1733–1748, https://doi.org/10.5194/cp-9-1733-2013, 2013.
Villagran, C.: Glacial climates and their efects on the history of the vegetation of Chile: a synthesis based on palynological evidence from Isla de Chiloe, Rev. Palaeobot. Palynol., 65, 17–24, 1990.
Weller, R., Minikin, A. Petzold, A., Wagenbach, D., and Konig-Langlo, G.: Characterization of long-term and seasonal variations of black carbon (BC) concentrations at Neumayer, Antarctica, Atmos. Chem. Phys., 13, 1579–1590, https://doi.org/10.5194/acp-13-1579-2013, 2013.
Westerling, A. L., Hidalgo, H. G., Cayan, D. R., and Swetnam, T. W.: Warming and earlier spring increase western US forest wildfire activity, Science, 313, 940–943, 2006.
Whitlock, C. and Larsen, C.: Charcoal as a fire proxy, in: Tracking Environmental Change Using Lake Sediments Terrestrial, Algal, and Siliceous Indicators, edited by: Smol, J. P., Birks, H. J. B., and Last, W. M., vol. 3. Kluwer Academic Publishers, Dordrecht, 75–97, 2002.
Whitlock C., Moreno P. I., and Bartlein P.: Climatic controls of Holocene fire patterns in southern South America, Quaternary Res., 68, 28–36 https://doi.org/10.1016/j.yqres.2007.01.012, 2007.
Wolff, E. and Cachier, H.: Concentration and seasonal cycle of black carbon in aerosol at a coastal Antarctic station, J. Geophys. Res., 103, 11033–11041, 1998.
WOOD, S. N.: Generalized additive models: an introduction with R. Boca Raton, FL, Chapman & Hall/CRC, 2006.
Yao, P., Schwab, V. F., Roth, V.-N., Xu, B., Yao, T., and Gleixner, G: Levoglucosan concentrations in ice-core samples from the Tibetan Plateau determined by reverse-phase high-performance liquid chromatography–mass spectrometry, J. Glaciol., 59, https://doi.org/10.3189/2013JoG12J157, 2013.
Zangrando, R., Barbaro, E., Zennaro, P., Rossi, S., Kehrwald, N. M., Gabrieli, J., Barbante, C., and Gambaro, A.: Molecular markers of biomass burning in Arctic aerosols, Environ. Sc. Technol., 47, 8565–8574, 2013.
Zangrando, R., Barbaro, E., Vecchiato, M., Kehrwald, N.M, Barbante, C., and Gambaro, A.: Levoglucosan and phenols in Antarctic marine, coastal and plateau aerosols, Sci. Total Environ., 544, 606–616, 2016.
Zennaro, P., Kehrwald, N., McConnell, J. R., Schüpbach, S., Maselli, O. J., Marlon, J., Vallelonga, P., Leuenberger, D., Zangrando, R., Spolaor, A., Borrotti, M., Barbaro, E., Gambaro, A., and Barbante, C.: Fire in ice: two millennia of boreal forest fire history from the Greenland NEEM ice core, Clim. Past, 10, 1905–1924, https://doi.org/10.5194/cp-10-1905-2014, 2014.
Zennaro, P., Kehrwald, N. M., Marlon, J., Ruddiman, W. F., Brücher, T., Agostinelli, C., Dahl-Jensen, D., Zangrando, R., Gambaro, A., and Barbante, C.: Europe on fire three thousand years ago: Arson or climate?, Geophys. Res. Lett., 42, 5023–5033, https://doi.org/10.1002/2015GL064259, 2015.
Zohary, D., Hopf, M., and Weiss, E.: Domestication of plants in the old world: The origin and spread of domesticated plants insouth-west Asia, Europe, and the Mediterranean basin, 4th Edn., Oxford University Press, Oxford, 2012.