the Creative Commons Attribution 4.0 License.
the Creative Commons Attribution 4.0 License.
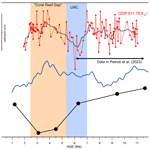
Impact of the Late Miocene Cooling on the loss of coral reefs in the Central Indo-Pacific
Benjamin F. Petrick
Lars Reuning
Miriam Pfeiffer
Gerald Auer
Lorenz Schwark
The Late Miocene Cooling (LMC) has been recognized as a global event in the climate record and posited as the start of modern ecosystems. Whereas climate shifts in modern tropical terrestrial ecosystems around 7.0–5.4 Ma are known, little is known about the impact of the LMC on coral reefs, where few good proxy records exist. During the Pliocene, a stratigraphic interval is present in the Central Indo-Pacific, where reefs that were present at the start of the Messinian disappeared by the Early Pliocene. This “Pliocene Reef Gap” has often been ascribed to non-climatic factors. However, a lack of proxy data prevents an understanding of climatic changes during this time. Here, we present a TEX-based sea surface temperature (SST) record for the Coral Sea, suggesting that the LMC is present across the Central Indo-Pacific. During the LMC, SST at ODP Site 811 declined by about 2 °C, while cooling lasted from 7.0 to 5.4 Ma. This cooling has also been seen in other parts of the Central Indo-Pacific. The LMC caused many changes in the Central Indo-Pacific, including a southwest shift in the monsoon belt, changes in terrestrial inputs, and changes in the strength of ocean currents. All of these factors can be stressors affecting coral reef growth. This suggests the overall impact of the LMC was to increase the stress on reef systems, which could have provided a driver for the collapse of individual reefs and therefore a potential cause for the Pliocene Reef Gap. The change in SST and other stressors associated with the cooling caused coral reef systems to collapse across the Central Indo-Pacific.
- Article
(3675 KB) - Full-text XML
-
Supplement
(462 KB) - BibTeX
- EndNote
1.1 LMC background
Global sea surface temperature (SST) records have identified the Late Miocene Cooling (LMC) as a worldwide event occurring between 7.0–5.4 Ma when SST globally decreased by about 6 °C (Herbert et al., 2016; Holbourn et al., 2018; Martinot et al., 2022; Tanner et al., 2020; Wen et al., 2023). However, the LMC does not occur in benthic δ18O stacks or splices (Westerhold et al., 2020; Zachos et al., 1994). It is a major climatic cooling that does not seem to be associated with any changes in ice volume or deep-water temperatures (Herbert et al., 2016; Martinot et al., 2022; Tanner et al., 2020). Therefore, it has often been overlooked as a low-latitude climatic factor impacting tropical marine ecosystems.
The LMC has been linked to aridification in Asia and Africa due to changes in the monsoonal system (Dupont et al., 2013; Feakins, 2013; Wen et al., 2023). This resulted in significant shifts from C3 to C4 plants in tropical zones, indicating the expansion of tropical grasslands (Huang et al., 2007; Steinthorsdottir et al., 2021; Strömberg and Strömberg, 2011). Due to grassland expansion in East Africa, it is thought that some of the earliest hominids began to evolve around 7 Ma to adapt to the changed climatic conditions (Brunet, 2020). In the ocean, a biogenic bloom marked by an increase in the δ13C of benthic foraminifera occurred just before the onset of the LMC at around 8 Ma (Diester-Haass et al., 2004; Drury et al., 2018; Lübbers et al., 2019).
1.2 Causes of the LMC
The causes of the LMC are not well understood. The paradox of a major cooling without a concurrent increase in glaciation has still not been fully explained. There are a series of short-term glacials, but these only occur after 6 Ma, almost 1 Myr after the onset of the cooling and at the start of the warming following the LMC (Jöhnck et al., 2020). Therefore, whatever triggered the LMC must have had little impact on the size of glaciers or deep-water temperatures. There are two primary explanations for the cooling associated with the LMC. These are gateway changes causing shifts in ocean circulation and changes in atmospheric CO2.
Ongoing re-organization in the Indonesian Throughflow (Hall, 2002, 2009; Gallagher et al., 2024) and the Isthmus of Panama (Collins et al., 1996; Haug et al., 2001) may offer a tectonic mechanism for the LMC due to the re-organization of the global thermohaline circulation. However, almost all known major thresholds in these systems date to the Pliocene or later (Auer et al., 2019; Haug et al., 2001; De Vleeschouwer et al., 2018, 2019), making these tectonic restrictions of oceanic gateways an unlikely cause. Furthermore, available SST data show that changes observed during the LMC eventually reversed after 5.4 Ma. This temporary and reversible pattern further emphasizes that long-term and permanent tectonic oceanic gateway closure could not have been the primary driver of the LMC.
An alternative hypothesis is that there was a reduction in atmospheric pCO2 during the LMC. Lower pCO2 would explain the difference between the increased tropical presence of C4 grasses, which were better adapted to lower atmospheric CO2 concentrations (Herbert et al., 2016; Wen et al., 2023). Model estimates, including atmospheric CO2 reductions, also fit well with temperature reconstructions, showing a cooling of about 2–3 °C in the Central Indo-Pacific (Martinot et al., 2022). The cause of the Late Miocene CO2 reduction is not well understood. One suggestion is that it was related to higher oceanographic productivity, which would likely draw down atmospheric CO2 (Holbourn et al., 2018). This could result from the biogenic bloom at 8–7 Ma (Diester-Haass et al., 2004; Grant and Dickens, 2002). Another theory is that changes in plate motion during the LMC led to a reduction in CO2 input to the atmosphere (Herbert et al., 2022). Finally, it has been suggested that the tectonic uplift of Papua New Guinea led to a long-term decrease in atmospheric CO2 (Martin et al., 2023; Clift et al., 2024).
1.3 Miocene reef history
There was an extensive coral reef system on the Queensland Plateau during the Early to Middle Miocene (Feary et al., 1991; Betzler and Chaproniere, 1993). After 11 Ma, however, the coral reefs appear to have retreated (Isern et al., 1993, 1996). Benthic foraminiferal reconstructions of sea level show a gradual increase in relative water depth due to increasing subsidence of the Queensland Plateau between 13–8 Ma (Katz and Miller, 1993). This roughly corresponds to the timing of the so-called Carbonate Crash, a period of low carbonate accumulation in the deep sea of all major tropical ocean basins (Lübbers et al., 2019). It was hypothesized that this episode also reduced carbonate accumulation rates in reefs in the South China Sea (Mathew et al., 2020). A similar mechanism could have made the reefs on the Queensland Plateau more susceptible to subsidence-related drowning and later collapse due to climatic changes. Other reefs in the Coral Sea and surrounding areas show evidence of a later collapse, many occurring between 7.0–5.4 Ma during the LMC. For instance, in the southern Coral Sea, coral reefs on Marion Plateau disappeared between 7.5–5.7 Ma (Bashah et al., 2024; Eberli et al., 2010; Ehrenberg et al., 2006). On the other side of Australia, the North West Shelf barrier reef experienced a major drowning event between 7.2–5.9 Ma (Belde et al., 2017; Rosleff-Soerensen et al., 2012, 2016). Finally, studies of Early Pliocene sediments on the Queensland Plateau show hardgrounds on the shallow carbonate platforms, suggesting a complete absence of coral reefs during the “Pliocene Reef Gap” (Droxler et al., 1993).
Globally, similar reef trends are seen. The highest abundance of coral reefs occurs in the Middle Miocene, followed by a slight decline towards the Late Miocene (Harrison et al., 2023; Perrin and Kiessling, 2012). However, by the Early Pliocene, there appears to have been a loss of coral reefs. This suggests that the major reef loss occurred between the beginning of the Messinian and the beginning of the Early Pliocene (7.25–5.33 Ma). Harrison et al. (2023) attribute reef loss in the Central Indo-Pacific to several factors impacting individual reefs differently (Fig. 2). These include tectonic processes, sea level changes, and increases in terrestrial input. The authors reject climate change as a causal factor, arguing that SSTs during the Late Miocene were similar to modern ones and that there is no evidence of major warming across this time (Harrison et al., 2023). However, there is evidence that the tropical water belt cooled and contracted during the LMC (Martinot et al., 2022; Liu et al., 2022). Moreover, there were changes in the global distribution of reefs during this time, including a decrease in their latitudinal range (Perrin and Kiessling, 2012).
1.4 Tropical SST change during the LMC
As discussed above, the climatic impact of the LMC has not been investigated as a driver of the Pliocene Reef Gap because many previous studies of benthic δ18O isotope records did not show an increase in glaciation during the LMC (Harrison et al., 2023; Perrin and Kiessling, 2012). Furthermore, even after the LMC was identified, many early tropical records showed less than 1 °C cooling during the LMC (Herbert et al., 2016). As a result, it was concluded that the temperature impact of the LMC in the tropics was small. However, this conclusion has been challenged as most LMC records are based on U-derived SST reconstructions (Herbert et al., 2016). These alkenone-based SST records have a saturation limit of 28 to 29 °C (Müller et al., 1998) when the proportion of the C37:3 isomer used for the temperature calculation approaches zero (Grimalt et al., 2001). Especially in carbonate rocks with low organic content and poor organic matter preservation, U SSTs are considered unreliable for temperatures exceeding 26–27 °C (Pelejero and Calvo, 2003; Grimalt et al., 2001). Models show that many parts of the tropical and sub-tropical Miocene Ocean were too warm for applying the U proxy (Burls et al., 2021). In fact, except ODP Site 722, all the sites where the U proxy was used to reconstruct SSTs are located in the cold tongue originating from the eastern Pacific equatorial upwelling zone (Herbert et al., 2016). ODP Site 722 is located in the Arabian Sea upwelling cell and is cooled by coastal upwelling driven by monsoonal winds (Bialik et al., 2020).
Mg/Ca and TEX86 records show a stronger cooling during the LMC. For instance, the western Pacific Warm Pool (WPWP) stack of TEX86 SSTs shows a cooling of 2 °C during the LMC (Liu et al., 2022). Furthermore, between 7–5 Ma, all the sites included in the WPWP stack show a cooling of 2–3 °C, suggesting a cooling of the entire WPWP (Liu et al., 2022; Zhang et al., 2014). This cooling is also confirmed by a Mg/Ca SST record from ODP Site 1146, one of the sites included in the WPWP TEX86 SST stack. In agreement with the TEX86 SSTs, the Mg/Ca SST shows a cooling of 2 °C (Holbourn et al., 2018). In the Indian Ocean, an Mg/Ca record from IODP Site 1443 shows a cooling of about 2 °C (Martinot et al., 2022). However, these records mainly derive from the northern margins of the Central Indo-Pacific warm-water area. Therefore, while the magnitude of cooling and timing of the LMC in these records is similar, it is unclear whether this was a large-scale, tropical-zone-wide event that could have affected coral reefs across the Central Indo-Pacific.
1.5 Objective
To better understand the link between tropical SSTs and the LMC, new records need to be produced from across the Central Indo-Pacific (Spalding et al., 2019; Crandall et al., 2019). In this study, we present a TEX SST record covering the LMC from ODP Site 811, located on the Queensland Plateau in the Coral Sea (Fig. 1). This is an extension of a record previously published in Petrick et al. (2023) that spanned the period from 6.6–11.1 Ma. In this study, we added new TEX SST data between 2.5 and 6.6 Ma to investigate the LMC and the Pliocene. We chose this site because the Coral Sea has one of the highest coral reef densities in the world (Bridge et al., 2019) and is bordered by the Great Barrier Reef off the eastern Australian coast. The Central Indo-Pacific is a hotspot of coral diversity during the Miocene and therefore a key reef area (Renema et al., 2008). There is abundant evidence of reef loss during the Late Miocene in the Coral Sea (Bashah et al., 2024; Betzler et al., 2024). This paper investigates whether climate change during the LMC could have led to the collapse of reef systems across the Central Indo-Pacific and globally.
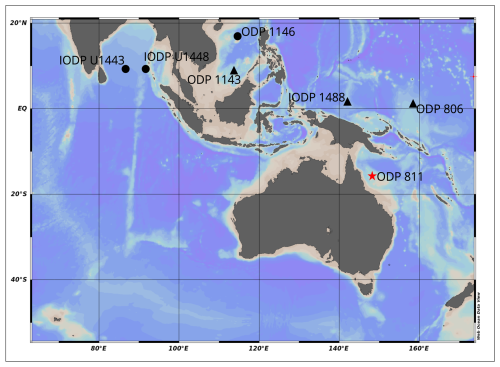
Figure 1Map showing the Central Indo-Pacific and the location of ODP Site 811 studied here (red star). All the sites included in the TEX86-derived western Pacific Warm Pool stack (Liu et al., 2022) are indicated with black triangles (Fig. 6). All Mg/Ca records are shown with black dots (Fig. 5). The base map is from Ocean Data View (Schlitzer, 2021).
Biogeochemistry
We solvent-extracted 50 cc of sediment for this project, which resulted in between 50 and 60 g of sediment, which is a suitable amount for obtaining sufficient lipids for TEX86 determination. Dried and homogenized samples were Soxhlet extracted for 48 h using a solvent mixture of DCM:MeOH (9:1, ). The addition of activated copper turnings removed elemental sulfur. A Büchi solvent evaporator reduced excess solvent to a final volume of 2 mL. Samples were then transferred into a 4 mL vial, where the total extract (TE) was taken to dryness under a gentle stream of nitrogen. TEs were fractionated into aliphatic, aromatic, and polar fractions by silica gel column chromatography (6 mL SPE column, 2.8 g silica gel 60 mesh, 25–40 µm) using solvents with increasing polarity in an LC-TECH automated SPE system. NSO (polar) compounds were eluted with 14 mL DCM / MeOH (). The polar fraction was reconstituted in hexane / isopropanol () and re-chromatographed over aminopropyl-substituted silica gel (3 mL SPE column, 1.0 g aminopropyl silica, 25–40 µ m). The alcohol fraction containing the glycerol dialkyl glycerol tetraethers (GDGTs) was eluted with 5 mL of hexane / isopropanol () and after drying was re-dissolved in hexane / isopropanol () to a final concentration of 6 mg mL−1 for injection into the HPLC/MS system.
GDGTs were measured on an AGILENT liquid chromatograph coupled to an AGILENT single-quadrupole mass spectrometer following the analytical protocol of Hopmans et al. (2016). The HPLC instrument was equipped with an AGILENT HILIC silica column (2.1 × 150 mm, 1.5 µm particle size) and a guard column maintained at 30 °C. Detection of archaeal core lipids was achieved by single ion recording of their protonated molecular ions [M + H+], and compounds were quantified by integration of peak areas using AGILENT Masshunter© software. Calculation of TEX followed Kim et al. (2010). Reproducibility upon duplicate measurements showed a relative standard error of < 2 %.
3.1 Site details
ODP Site 811 is located in the Coral Sea (16.516° S, 148.157° E) and has a modern water depth of 937.0 m (Fig. 1). Benthic foraminifera data show that the site was probably shallower during the start of the Late Miocene (< 500 m) and that the site only reached its current depth around the Early Pliocene (Katz and Miller, 1993). For this study, we focused on the record from Hole A between 113 and 27 m. The age model from this site was created using a new nannofossil stratigraphic study. This was published by Petrick et al. (2023), including the data and errors involved. The studied interval consists almost entirely of nannofossil to foraminiferal ooze. In the upper 70 m b.s.f. (meters below the sea floor), the pelagic components are mixed with fine shallow-water bank-derived particles. The sediment was interpreted as periplatform ooze (Fig. 2). One debris flow occurs close to the top of the studied interval (811A-4H-6), which was avoided during sampling (Fig. 2). The interval between 70 and 113 m b.s.f. is characterized by purely pelagic sediments (Fig. 2). The carbonate content in the studied section always exceeds 90 wt %. None of these lithologic boundaries match changes in the TEX86 data or the timing of the LMC (Fig. 2). The modern annual average SST at Site 811 is 26.1 °C (Locarnini et al., 2019). The average summer temperature is 28.32 °C, and the average winter temperature is 25.09 °C (Locarnini et al., 2019).
3.2 TEX86 tests
The new TEX record for 1.9–6.6 Ma complements previous data covering 6.6 to 11.1 Ma (Petrick et al., 2023). The data for this study and data from Petrick et al. (2023) are presented in Fig. S1 in the Supplement. Several tests were performed to evaluate the new data and ensure that it reflects SST changes and is not the result of nonthermal factors impacting GDGTs. With only a few exceptions, the TEX data passed all applied tests. We will discuss the various tests, what they show, and why we decided to remove (or retain) data points.
The methane index (MI) excludes any data affected by gas-hydrate-related anaerobic methane oxidation (Zhang et al., 2011). The MI values are below the 0.5 value recommended for rejection (Fig. 3a). We also used the %GDGT-0 index to eliminate samples with GDGTs substantially originating from sedimentary archaeal methanogenesis (Weijers et al., 2006; Sinninghe Damsté et al., 2012). The values were well below the 67 % cut-off for excessive methanogenesis (Fig. 3b). We used the ring index (RI) to evaluate whether the GDGTs deviate from modern values (Zhang et al., 2016). All of our data fell within the acceptable error envelope of 0.3 (Fig. 3d). Finally, we used the index to ensure that the GDGTs were being formed near the surface (Taylor et al., 2013; Hernández-Sánchez et al., 2014). This measures the relationship between the compounds GDGT 2 and GDGT 3, which is related to the depth of production of archaeal GDGTs. While the appropriate cut-off for this test is still being debated, the data values are low (< 5) and indicate surface production (Rattanasriampaipong et al., 2022).
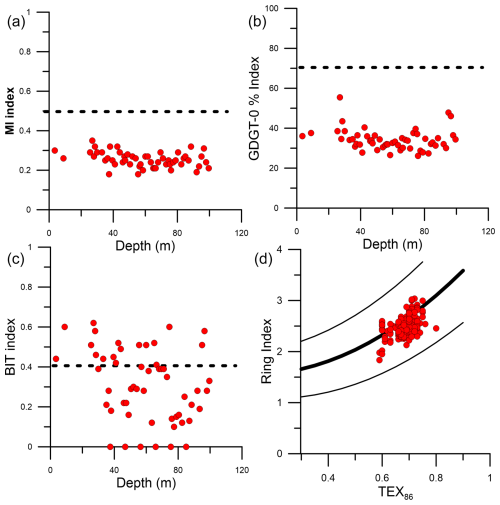
Figure 3Graphical illustration of the new ODP Site 811 data for various GDGT indices used for quality assurance for previously published data (Petrick et al., 2023). Quality criteria shown are the (a) methane index (MI, after Zhang et al., 2011), (b) %GDGT-0 index (after Sinninghe Damsté et al., 2012), (c) branched vs. isoprenoid index (BIT, proposed by Schouten et al., 2002), and (d) ring index (RI, proposed by Zhang et al., 2016). Quality assurance tests indicate that GDGT data are suitable for SST reconstruction and are not compromised by other environmental drivers.
One GDGT index that did not fit recommendations for quality assurance was the branched vs. isoprenoid index (BIT, Fig. 3c). This index was developed to track the amount of terrigenous material that could interfere with the TEX86 values via soil-sourced GDGTs (Schouten et al., 2013). The original cut-off point is 0.4, although there is a debate about whether that cut-off is too strict (Schouten et al., 2013) and how to evaluate variations in crenarchaeol shown to affect the BIT index (Fietz et al., 2011). Although 11 samples exceed a BIT value of 0.5 (Fig. 3c) within the GDGT suite analyzed here, the data show no covariance with either time, depth, or SST (Fig. S1). The highest BIT values do not match the highest or lowest SST data. Finally, removing the high BIT samples does not affect the trends or conclusions of the paper. Therefore, we have decided to retain all data in the results based on the current debate regarding the reliability of the BIT index.
3.3 SST trends
For this study, we follow the age model of Petrick et al. (2023), which updated the original shipboard (Davies et al., 1991) and a previously published age model (Isern et al., 1993, 1996) (Fig. 2). A part of the record presented in this study (6.6–11.1 Ma) was previously published in Petrick et al. (2023) and is discussed in more detail in Sect. 4.1 (Fig. 4). It is also important to note that the ODP Site 811 SST record has a lower resolution than other nearby records. This may mean that internal variability within the record could exaggerate the LMC cooling trend. Therefore, we used both a running average and a fixed time window for the LMC to quantify the amount of cooling in the record. However, the boundaries of the LMC are uncertain. We therefore estimated LMC cooling using two different definitions of the LMC (Table 1). First, we compared SSTs before the LMC (11.1–7.0 Ma) to the LMC, following Herbert et al. (2016), where the LMC is defined as 7.0–5.4 Ma. We then used the windows used in Martinot et al. (2022) for the pre-LMC (8.5–7.5 Ma) and the maximum of the LMC (6.5–5.5 Ma). The data are summarized in Table 1. The average cooling at ODP Site 811 during the LMC is 2 ± 0.2 °C across all windows. We will use 2 °C as our best estimate of LMC cooling at Site 811.
Table 1Different definitions of the LMC compared in this study, average SSTs, and LMC cooling at Site 811.

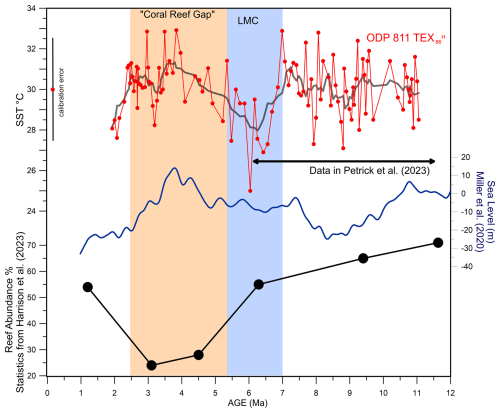
Figure 4The TEX-derived SST record for ODP Site 811 is shown in red, including data from 6.6 to 11.1 Ma taken from Petrick et al. (2023). The gray line represents an eight-point running average. The calibration error from Kim et al. (2010) is shown at the top. The sea level evolution is shown in blue after Miller et al. (2020). The relative abundance (%) of areas covered by reefs in the Central Indo-Pacific is indicated by the black line and dots at the bottom, with data taken from Harrison et al. (2023). Note that we have followed Harrison et al. (2023) by setting the reef abundance percentage for each faunal stage at the midpoint of the stage. The exception is the value of the Serravallian, which is shown at the end of the period (11.63 Ma) to fit it into the figure.
4.1 Previous work at ODP 811
The JOIDES Resolution drilled ODP Site 811 as part of Expedition 133 in the Coral Sea (Davies et al., 1991) (Fig. 1). ODP Site 811 was located further south during the Late Miocene (likely ∼ 19.4 to 17.5° S) (Van Hinsbergen et al., 2015). As part of the post-cruise work, a δ18O record was produced using planktonic foraminifera, which showed SSTs were between 18–24 °C during the Late Miocene (Isern et al., 1993, 1996). These cold temperatures were proposed to be the reason for the collapse of the coral reefs in the Coral Sea between 11–8 Ma (Isern et al., 1993, 1996). However, many similar foraminiferal δ18O records showing cool tropical SSTs have been re-evaluated subsequently using biomarkers and well-preserved foraminifera. These studies show that post-depositional alteration of calcite is a larger source of error than originally thought (Nairn et al., 2021; Wilson et al., 2002). Petrick et al. (2023) used TEX to reconstruct SSTs over the 8–12 Ma period when the reefs in the Coral Sea drowned (Isern et al., 1993) (Fig. 4). This record showed an average SST of 30 °C between 8–12 Ma. Petrick et al. (2023) concluded that a combination of low aragonite saturation and heat stress impaired coral growth and that relative sea level rise combined with these stressors and potential changes in ocean circulation led to the drowning of the coral reefs (Petrick et al., 2023). Therefore, the period of major reef loss occurred before the LMC at ODP Site 811 (Isern et al., 1993, 1996). However, other major reef systems in the Coral Sea did drown between 7–5.4 Ma, including the Marion Plateau (Bashah et al., 2024). Therefore, while ODP Site 811 is not located in an area that experienced coral reef loss during the LMC, it can still be used to understand the climatic impact of the LMC on the Coral Sea and the wider Central Indo-Pacific.
4.2 ODP Site 811 and the LMC
As shown above, the cooling at ODP Site 811 during the LMC is about 2 °C (Fig. 4). This is consistent with other records from the Central Indo-Pacific, including ODP Site 1146 and IODP Site U1448 (Holbourn et al., 2018; Martinot et al., 2022) (Fig. 5). This also matches the magnitude of cooling seen in TEX86 records from the WPWP (Liu et al., 2022; Zhang et al., 2014) (Fig. 6). Therefore, the new ODP Site 811 data confirm previous findings (Holbourn et al., 2018; Liu et al., 2022; Martinot et al., 2022), showing that the cooling of the tropics was greater than the 0.5 °C during the LMC, as initially suggested by Herbert et al. (2016). Furthermore, the data suggest that the cooling in the Central Indo-Pacific was uniform during the LMC.
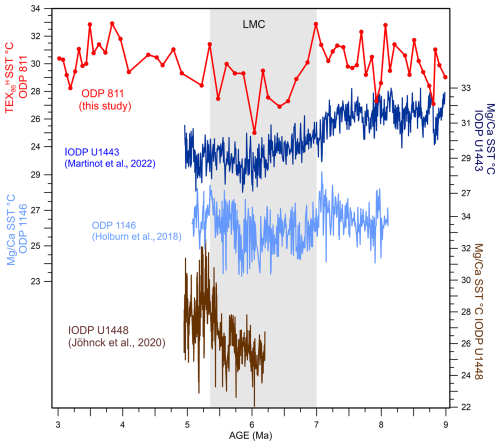
Figure 5ODP Site 811 TEX-derived SSTs compared to the Mg/Ca-derived SST records. The gray bar marks the LMC as defined in Herbert et al. (2016). Sites are shown in Fig. 1.
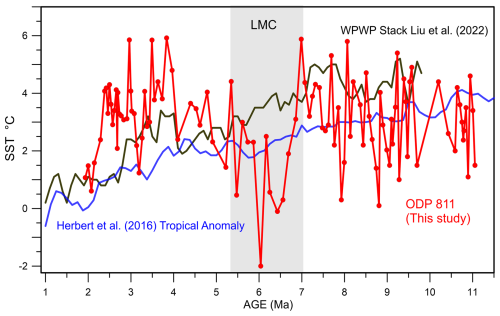
Figure 6ODP Site 811 (red) temperature anomalies compared to the tropical anomaly (blue) from Herbert et al. (2016) and the WPWP stack (black) (Liu et al., 2022). SST data for this plot have been normalized to modern temperatures to better compare with the anomaly data presented by Herbert et al. (2016). This was done for ODP Site 811 and the WPWP stack by subtracting modern SSTs from the data. The gray bar delineates the LMC as defined by Herbert et al. (2016). Sites included in the WPWP stack are shown in Fig. 1.
Unfortunately, our records can only provide limited information regarding the causes of LMC. The ODP Site 811 temperature trends are consistent with the expected cooling inferred using climate modeling (Martinot et al., 2022), which estimates SSTs of around 28 °C and a cooling of about 2 °C at ODP Site 811 when atmospheric CO2 is lowered to 280 ppm. Given that ODP Site 811 and IODP Site 1448 are both located in the Central Indo-Pacific warm pool but in different ocean basins and hemispheres, the fact that mean SSTs and LMC cooling are consistent with climate models could strengthen the argument that a change in atmospheric CO2 caused the LMC. Therefore, our ODP Site 811 record suggests that changes in pCO2 may be responsible for the cooling seen in the record.
The major difference between the ODP Site 811 record and other records from the Central Indo-Pacific seems to be the post-cooling recovery of temperatures (Figs. 5, 6). Not all sites return to pre-LMC temperatures. The timing of post-LMC recovery is also different between sites. ODP Site 811 remained cool after the LMC but fully recovered by the Early Pliocene–Late Pliocene boundary (Fig. 4). Other records show a dramatic post-LMC recovery, such as IODP Site 1448 (Jöhnck et al., 2020) between 5.5–5 Ma (Fig. 5).
In contrast, the TEX86 WPWP stack shows a long-term cooling trend and no clear recovery after the LMC, although there is warming around 4 Ma (Liu et al., 2022) (Fig. 6). Therefore, while the Central Indo-Pacific experienced the same 2–3 °C cooling during the LMC, the recovery was heterogeneous (Figs. 5, 6). We conclude that if the Pliocene Reef Gap was triggered by climatic change, it was most likely the LMC cooling and not the post-LMC recovery.
4.3 Potential stressors in the Central Indo-Pacific
As shown above, ODP Site 811 experienced a rapid and strong cooling associated with the LMC (Fig. 2). Furthermore, SSTs did not recover until at least 5 Ma, well into the Pliocene Reef Gap. The cooling was widespread, as it was recorded at many sites in the Central Indo-Pacific. Therefore, it could have negatively impacted coral reefs and carbonate platform ecosystems. Studies show that globally, there was a reduction in the latitudinal extent of coral reefs during the Late Miocene, including the late LMC (Perrin and Kiessling, 2012). On the Marion Plateau, south of ODP Site 811, reefs disappeared around 7 Ma (Bashah et al., 2024; Ehrenberg et al., 2006; Isern et al., 2004). Today, an SST gradient of about 4 °C exists between ODP Site 811 and the southern Marion Plateau (Locarnini et al., 2019). ODP Site 811 had average SSTs of about 28 °C and possibly as cold as 24 °C during the LMC. Therefore, SSTs could have been as cold as 20 °C on the southern Marion Plateau. Coral reefs have lower carbonate accumulation due to lower coral growth rates at these temperatures (Laugié et al., 2019; Lough, 2008; Lough and Barnes, 2000; Lough and Cantin, 2014). This can impair their ability to adapt to changing oceanographic conditions (Higuchi et al., 2015). Other key carbonate producers associated with reefs show a similar temperature dependence during the Miocene (Bassi et al., 2024). Porcelaneous larger benthic foraminifera (pLBF) diversity is at its lowest level during the Messinian (almost contemporaneous with the LMC), with only three species identified for the Central Indo-Pacific (Bassi et al., 2024). Since pLBF are a crucial contributor to carbonate production in Miocene coral reef environments, this decrease could have negatively impacted the accretion rate of the reef. Thus, the cold SSTs during the LMC could have negatively impacted many of these higher-latitude coral reefs and contributed to the latitudinal contraction of coral reefs (Perrin and Kiessling, 2012).
However, as discussed in Petrick et al. (2023), stressors other than SSTs can affect the coral reefs. A larger latitudinal SST gradient during the LMC may have been associated with changes in the ocean circulation, as seen during other major cooling events (Petrick et al., 2018, 2019). It has already been proposed that changes in the strength of ocean currents could have caused greater erosion of carbonate platforms, possibly impacting the expansion of coral reefs (Betzler et al., 2024; Betzler and Eberli, 2019). There is some evidence of stronger ocean currents during the LMC in the Central Indo-Pacific. On the Marion Plateau, between 7.5 and 5.7 Ma, the intensification of the East Australian Current caused an increase in drift deposits (Bashah et al., 2024; John and Mutti, 2005). On the North West Shelf, there is evidence of an intensification of NNE–SSW-oriented bottom currents starting in the Late Miocene after 7.2 Ma (Thronberens et al., 2022), contributing to the demise of the regional reef system (Belde et al., 2017). Therefore, a similar Central Indo-Pacific-wide strengthening of ocean currents could have occurred during the LMC.
Changes in terrestrial input could also result from shifts in SSTs. There is evidence that SST cooling during the Late Miocene led to southward shifts in the rain belts (Jöhnck et al., 2020). This may have increased rainfall and terrestrial input in northern Australia and the Indonesian archipelago (Jöhnck et al., 2020; Tagliaro et al., 2018). Finally, in the Coral Sea, the weathering of Papua New Guinea intensified, and there is evidence of increased terrestrial input to the northern Coral Sea from DSDP Sites 210 and 287 (Clift et al., 2024). During the Miocene, many coral reefs in the Indonesian archipelago grew in turbid environments (Santodomingo et al., 2016). However, increases in sediment input could have upset the delicate balance on which these corals depended. Therefore, changing rainfall patterns during the LMC could have been an additional stressor for the coral reefs.
Therefore, the LMC was associated with major environmental changes that could have acted as stressors impacting the coral reefs, causing widespread drowning. Previous work in the southern Coral Sea has shown that similar changes in temperature, terrestrial input, and ocean current led to changes in the benthic assemblage of carbonate producers (Hallock et al., 2006). This modified the accretion potential of the carbonate platforms and contributed to their drowning in the Miocene (Hallock et al., 2006). This is also similar to modern coral systems, where current temperature changes are accompanied by multiple other environmental stressors, such as sea level rise and increased sediment loads (Cornwall et al., 2021). While coral reefs might be able to adapt to single stressors, such as higher sea levels, multiple stressors may add up synergistically and trigger coral reef collapse (Darling and Côté, 2013).
Finally, while the LMC cooling was unrelated to a sea level change, the global sea level increased after 5 Ma (Miller et al., 2020) (Fig. 4). As a result, the carbonate platform tops were no longer in the photic zone when SSTs eventually returned to the Middle Miocene levels during the Middle Pliocene. As a result, the corals could not grow back. It was noted that the re-establishment of coral reefs in the Coral Sea was linked to a global sea level drop at ∼ 2.9 Ma, which would have brought the platforms back into the photic zone, allowing coral reefs to develop again (Droxler et al., 1993) (Fig. 4). Therefore, it is likely that after the drowning during the LMC, the reestablishment of the reefs was more related to changes in sea level than SST.
The new TEX-derived SST data at ODP Site 811 show that the LMC led to a relatively rapid drop in SSTs by about 2 °C in the southern part of the Central Indo-Pacific reef province. This is consistent with other records from the Central Indo-Pacific, which show a 2–3 °C drop in SSTs at 7–6 Ma. This decline in tropical SST preceded the Pliocene Reef Gap and could have been a stress factor contributing to the loss of coral reefs in the Central Indo-Pacific reef province. It is probably responsible for the loss of reefs on the Southern Marion Plateau. Additionally, there is evidence that the changes in global and regional SSTs caused by the LMC led to shifts in ocean currents, as well as rain belts. Together with the cooling of SST, these multiple stressors may explain some of the changes seen previously in individual Upper Miocene reefs and provide an overall explanation for the region-wide coral reef decline over such a short time interval. This likely explains the massive reduction in the extent of coral reefs in the Pliocene Reef Gap. Therefore, this study emphasizes the detrimental impacts of climate change on coral reefs and the importance of limiting additional stressors, such as pollution, on reef ecosystems during global temperature change.
The data for this paper are available both in the Supplement and from the EU Open Research Europe Zenodo repository (https://doi.org/10.5281/zenodo.10902264, Petrick et al., 2024).
The supplement related to this article is available online at: https://doi.org/10.5194/cp-21-405-2025-supplement.
All authors approved the manuscript and agreed to its submission. All authors discussed the results and provided significant input on the final version of the manuscript. BP and LR designed the study. BP ran the project and processed the samples. LS performed the biomarker analysis in his lab and interpreted data with BP GA, who provided a new age model for the site. BP, LS, LR, MP, and GA provided vital feedback on the article.
The contact author has declared that none of the authors has any competing interests.
Publisher’s note: Copernicus Publications remains neutral with regard to jurisdictional claims made in the text, published maps, institutional affiliations, or any other geographical representation in this paper. While Copernicus Publications makes every effort to include appropriate place names, the final responsibility lies with the authors.
The authors would like to thank the participants of ODP Expedition 133 for recovering the core and providing the initial data and many of the studies used to reconstruct conditions in the Coral Sea. We thank the IODP core repository at Kochi for providing the samples. IODP provided critical support to this projectẆe thank Erin McClymont, Andre Droxler, and Christian Betlzer for their help and feedback on the paper.
This project was funded with a grant from the Deutsche Forschungsgemeinschaft (DFG) via project no. 447611930 awarded to Benjamin Petrick and contributes towards an Austrian Science Fund project (grant no. P 36046-N) awarded to Gerald Auer.
This paper was edited by Laurie Menviel and reviewed by three anonymous referees.
Auer, G., De Vleeschouwer, D., Smith, R. A., Bogus, K., Groeneveld, J., Grunert, P., Castañeda, I. S., Petrick, B., Christensen, B., Fulthorpe, C., Gallagher, S. J., and Henderiks, J.: Timing and Pacing of Indonesian Throughflow Restriction and Its Connection to Late Pliocene Climate Shifts, Paleoceanogr. Paleoclimatol., 34, 635–657, https://doi.org/10.1029/2018PA003512, 2019.
Bashah, S., Eberli, G. P., and Anselmetti, F. S.: Archive for the East Australian current: carbonate contourite depositional system on the Marion Plateau, Northeast Australia, Mar. Geol., 463, 107224, https://doi.org/10.1016/j.margeo.2024.107224, 2024.
Bassi, D., Braga, J. C., Pignatti, J., Fujita, K., Nebelsick, J. H., Renema, W., and Iryu, Y.: Porcelaneous larger foraminiferal responses to Oligocene–Miocene global changes, Palaeogeogr. Palaeocl., 634, 111916, https://doi.org/10.1016/j.palaeo.2023.111916, 2024.
Belde, J., Back, S., Bourget, J., and Reuning, L.: Oligocene and Miocene Carbonate Platform Development In the Browse Basin, Australian Northwest Shelf, J. Sediment. Res., 87, 795–816, https://doi.org/10.2110/jsr.2017.44, 2017.
Betzler, C. and Chaproniere, G. C. H.: Paleogene and Neogene Larger Foraminifers from the Queensland Plateau: Biostratigraphy and Environmental Significance, Proceedings of the Ocean Drilling Program, 133 Scientific Results, https://doi.org/10.2973/ODP.PROC.SR.133.210.1993, 1993.
Betzler, C. and Eberli, G. P.: Miocene start of modern carbonate platforms, Geology, 47, 771–775, https://doi.org/10.1130/G45994.1, 2019.
Betzler, C., Brachert, T. C., and Kroon, D.: Role of climate in partial drowning of the Queensland Plateau carbonate platform (northeastern Australia), Mar. Geol., 123, 11–32, https://doi.org/10.1016/0025-3227(95)80002-S, 1995.
Betzler, C., Hübscher, C., Lindhorst, S., Lüdmann, T., Hincke, C., Beaman, R. J., and Webster, J. M.: Seismic stratigraphic and sedimentary record of a partial carbonate platform drowning, Queensland Plateau, north-east Australia, Mar. Geol., 470, 107255, https://doi.org/10.1016/j.margeo.2024.107255, 2024.
Bialik, O. M., Auer, G., Ogawa, N. O., Kroon, D., Waldmann, N. D., and Ohkouchi, N.: Monsoons, Upwelling, and the Deoxygenation of the Northwestern Indian Ocean in Response to Middle to Late Miocene Global Climatic Shifts, Paleoceanogr. Paleoclimatol., 35, 2, https://doi.org/10.1029/2019PA003762, 2020.
Bridge, T. C. L., Beaman, R. J., Bongaerts, P., Muir, P. R., Ekins, M., and Sih, T.: The Great Barrier Reef and Coral Sea, Springer, Cham, 351–367, https://doi.org/10.1007/978-3-319-92735-0_20, 2019.
Brunet, M.: Sahelanthropus tchadensis dit “Toumaï”: le plus ancien membre connu de notre tribu, Bull. Acad. Natl. Med., 204, 251–257, https://doi.org/10.1016/j.banm.2019.12.017, 2020.
Burls, N. J., Bradshaw, C. D., Boer, A. M. De, Herold, N., Huber, M., Pound, M., Donnadieu, Y., Farnsworth, A., Frigola, A., Gasson, E., Heydt, A. S. von der, Hutchinson, D. K., Knorr, G., Lawrence, K. T., Lear, C. H., Li, X., Lohmann, G., Lunt, D. J., Marzocchi, A., Prange, M., Riihimaki, C. A., Sarr, A.-C., Siler, N., and Zhang, Z.: Simulating Miocene Warmth: Insights From an Opportunistic Multi-Model Ensemble (MioMIP1), Paleoceanogr. Paleoclimatol., 36, e2020PA004054, https://doi.org/10.1029/2020PA004054, 2021.
Clift, P. D., Du, Y., Mohtadi, M., Pahnke, K., Sutorius, M., and Böning, P.: The erosional and weathering response to arc–continent collision in New Guinea, J. Geol. Soc. London, 181, 4, https://doi.org/10.1144/jgs2023-207, 2024.
Collins, L. S., Coates, A. G., Berggren, W. A., Aubry, M.-P. M. P., and Zhang, J.: The late Miocene Panama isthmian strait, Geology, 24, 687–690, 1996.
Cornwall, C. E., Comeau, S., Kornder, N. A., Perry, C. T., van Hooidonk, R., DeCarlo, T. M., Pratchett, M. S., Anderson, K. D., Browne, N., Carpenter, R., Diaz-Pulido, G., D'Olivo, J. P., Doo, S. S., Figueiredo, J., Fortunato, S. A. V., Kennedy, E., Lantz, C. A., McCulloch, M. T., González-Rivero, M., Schoepf, V., Smithers, S. G., and Lowe, R. J.: Global declines in coral reef calcium carbonate production under ocean acidification and warming, P. Natl. Acad. Sci. USA, 118, 21, https://doi.org/10.1073/pnas.2015265118, 2021.
Crandall, E. D., Riginos, C., Bird, C. E., Liggins, L., Treml, E., Beger, M., Barber, P. H., Connolly, S. R., Cowman, P. F., DiBattista, J. D., Eble, J. A., Magnuson, S. F., Horne, J. B., Kochzius, M., Lessios, H. A., Liu, S. Y. V., Ludt, W. B., Madduppa, H., Pandolfi, J. M., Toonen, R. J., Contributing Members of the Diversity of the Indo-Pacific Network, and Gaither, M. R.: The molecular biogeography of the Indo-Pacific: Testing hypotheses with multispecies genetic patterns, Global Ecol. Biogeogr., 28, 943–960, https://doi.org/10.1111/geb.12905, 2019.
Darling, E. S. and Côté, I. M.: Vulnerability of Coral Reefs, edited by: Pielke, R. A. B. T.-C. V., Academic Press, Oxford, 259–270, https://doi.org/10.1016/B978-0-12-384703-4.00427-5, 2013.
Davies, P. J., Mackenzie, J. A., and Palmer-Julson, A.: Site 811–826 Northeast Australian Margin, in: Proc. ODP Init. Rep., Vol. 133, p. 810, https://doi.org/10.2973/odp.proc.ir.133.1991, 1991.
De Vleeschouwer, D., Auer, G., Smith, R., Bogus, K., Christensen, B., Groeneveld, J., Petrick, B., Henderiks, J., Castañeda, I. S., O'brien, E., Ellinghausen, M., Gallagher, S. J., Fulthorpe, C. S., and Pälike, H.: The amplifying effect of Indonesian Throughflow heat transport on Late Pliocene Southern Hemisphere climate cooling, Earth Planet. Sc. Lett., 500, 15–27, https://doi.org/10.1016/j.epsl.2018.07.035, 2018.
De Vleeschouwer, D., Petrick, B. F., and Martínez-García, A.: Stepwise Weakening of the Pliocene Leeuwin Current, Geophys. Res. Lett., 46, 8310–8319, https://doi.org/10.1029/2019GL083670, 2019.
Diester-Haass, L., Meyers, P. A., and Bickert, T.: Carbonate crash and biogenic bloom in the late Miocene: Evidence from ODP Sites 1085, 1086, and 1087 in the Cape Basin, southeast Atlantic Ocean, Paleoceanography, 19, 1007, https://doi.org/10.1029/2003PA000933, 2004.
Droxler, A. W., Haddad, G. A., Kroon, D., Gartner, S., Wei, W., and McNeill, D.: Late Pliocene (2.9 Ma) partial recovery of shallow carbonate banks on the Queensland Plateau: signal of bank-top reentry into the photic zone during a lowering in sea level, Proc., scientific results, ODP, Leg 133, northeast Australian margin, 235–254, https://doi.org/10.2973/ODP.PROC.SR.133.227.1993, 1993.
Drury, A. J., Lee, G. P., Gray, W. R., Lyle, M., Westerhold, T., Shevenell, A. E., and John, C. M.: Deciphering the state of the late Miocene to early Pliocene equatorial Pacific, Paleoceanogr. Paleoclimatol., 33, 246–263, https://doi.org/10.1002/2017PA003245, 2018.
Dupont, L. M., Rommerskirchen, F., Mollenhauer, G., and Schefuß, E.: Miocene to Pliocene changes in South African hydrology and vegetation in relation to the expansion of C4 plants, Earth Planet Sc. Lett., 375, 408–417, https://doi.org/10.1016/j.epsl.2013.06.005, 2013.
Eberli, G. P., Anselmetti, F. S., Isern, A. R., and Delius, H.: Timing of Changes in Sea-Level and Currents along Miocene Platforms on the Marion Plateau, Australia, in: Cenozoic Carbonate Systems of Australasia, SEPM (Society for Sedimentary Geology), 219–242, https://doi.org/10.2110/sepmsp.095.219, 2010.
Ehrenberg, S. N. N., McArthur, J. M. M., and Thirlwall, M. F. F.: Growth, Demise, and Dolomitization of Miocene Carbonate Platforms on the Marion Plateau, Offshore NE Australia, J. Sediment. Res., 76, 91–116, https://doi.org/10.2110/jsr.2006.06, 2006.
Feakins, S. J.: Pollen-corrected leaf wax D/H reconstructions of northeast African hydrological changes during the late Miocene, Palaeogeogr. Palaeocl., 374, 62–71, https://doi.org/10.1016/j.palaeo.2013.01.004, 2013.
Feary, D. A., Davies, P. J., Pigram, C. J., and Symonds, P. A.: Climatic evolution and control on carbonate deposition in northeast Australia, Palaeogeogr. Palaeocl., 89, 341–361, https://doi.org/10.1016/0031-0182(91)90171-M, 1991.
Fietz, S., Martínez-Garcia, A., Huguet, C., Rueda, G., and Rosell-Melé, A.: Constraints in the application of the Branched and Isoprenoid Tetraether index as a terrestrial input proxy, J. Geophys. Res., 116, C10032, https://doi.org/10.1029/2011JC007062, 2011.
Gallagher, S. J., Auer, G., Brierley, C. M., Fulthorpe, C. S., and Hall, R.: Cenozoic History of the Indonesian Gateway, Annu. Rev. Earth Pl. Sc., 52, 581–604, https://doi.org/10.1146/annurev-earth-040722-111322, 2024.
Grant, K. M. and Dickens, G. R.: Coupled productivity and carbon isotope records in the southwest Pacific Ocean during the late Miocene–early Pliocene biogenic bloom, Palaeogeogr. Palaeocl., 187, 61–82, https://doi.org/10.1016/S0031-0182(02)00508-4, 2002.
Grimalt, J. O., Calvo, E., and Pelejero, C.: Sea surface paleotemperature errors in U estimation due to alkenone measurements near the limit of detection, Paleoceanography, 16, 226–232, https://doi.org/10.1029/1999PA000440, 2001.
Groeneveld, J., Henderiks, J., Renema, W., McHugh, C. M., De Vleeschouwer, D., Christensen, B. A., Fulthorpe, C. S., Reuning, L., Gallagher, S. J., Bogus, K., Auer, G., Ishiwa, T., and Expedition 356 Scientists: Australian shelf sediments reveal shifts in Miocene Southern Hemisphere westerlies, Sci. Adv., 3, e1602567, https://doi.org/10.1126/sciadv.1602567, 2017.
Hall, R.: Southeast Asia's changing palaeogeography, Blumea – Biodiversity, Evolution and Biogeography of Plants, 54, 148–161, https://doi.org/10.3767/000651909X475941, 2009.
Hall, R.: Cenozoic geological and plate tectonic evolution of SE Asia and the SW Pacific: computer-based reconstructions, model and animations, J. Asian Earth Sci., 20, 353–431, https://doi.org/10.1016/S1367-9120(01)00069-4, 2002.
Hallock, P., Sheps, K., Chapronière, G., and Howell, M.: Larger benthic foraminifers of the Marion Plateau, northeastern Australia (ODP Leg 194): comparison of faunas from bryozoan (Sites 1193 and 1194) and red algal (Sites 1196–1198) dominated carbonate platforms, edited by: Anselmetti, F. S., Isern, A. R., Blum, P., and Betzler, C., Proc. ODP, Sci. Results, 194, 31 pp., https://doi.org/10.2973/odp.proc.sr.194.009.2006, 2006.
Harrison, G. W. M., Santodomingo, N., Johnson, K. G., and Renema, W.: Is the Coral Triangle's future shown in a Pliocene reef gap?, Coral Reefs, 42, 1219–1225, https://doi.org/10.1007/s00338-023-02412-5, 2023.
Haug, G. H., Tiedemann, R., Zahn, R., and Ravelo, A. C.: Role of Panama uplift on oceanic freshwater balance, Geology, 29, 207–210, 2001.
Herbert, T. D., Lawrence, K. T., Tzanova, A., Peterson, L. C., Caballero-Gill, R., and Kelly, C. S.: Late Miocene global cooling and the rise of modern ecosystems, Nat. Geosci., 9, 843–847, https://doi.org/10.1038/ngeo2813, 2016.
Herbert, T. D., Dalton, C. A., Liu, Z., Salazar, A., Si, W., and Wilson, D. S.: Tectonic degassing drove global temperature trends since 20 Ma, Science, 377, 116–119, 2022.
Hernández-Sánchez, M. T., Woodward, E. M. S., Taylor, K. W. R., Henderson, G. M., and Pancost, R. D.: Variations in GDGT distributions through the water column in the South East Atlantic Ocean, Geochim. Cosmochim. Ac., 132, 337–348, https://doi.org/10.1016/j.gca.2014.02.009, 2014.
Higuchi, T., Agostini, S., Casareto, B. E., Suzuki, Y., and Yuyama, I.: The northern limit of corals of the genus Acropora in temperate zones is determined by their resilience to cold bleaching, Sci. Rep., 5, 18467, https://doi.org/10.1038/srep18467, 2015.
Holbourn, A. E., Kuhnt, W., Clemens, S. C., Kochhann, K. G. D., Jöhnck, J., Lübbers, J., and Andersen, N.: Late Miocene climate cooling and intensification of southeast Asian winter monsoon, Nat. Commun., 9, 1584, https://doi.org/10.1038/s41467-018-03950-1, 2018.
Hopmans, E. C., Schouten, S., and Sinninghe Damsté, J. S.: The effect of improved chromatography on GDGT-based palaeoproxies, Org. Geochem., 93, 1–6, https://doi.org/10.1016/j.orggeochem.2015.12.006, 2016.
Huang, Y., Clemens, S. C., Liu, W., Wang, Y., and Prell, W. L.: Large-scale hydrological change drove the late Miocene C4 plant expansion in the Himalayan foreland and Arabian Peninsula, Geology, 35, 531–534, https://doi.org/10.1130/G23666A.1, 2007.
Isern, A. R., McKenzie, J. A., and Müller, D. W.: Paleoceanographic Changes and Reef Growth off the Northeastern Australian Margin: Stable Isotopic Data from ODP Leg 133 Sites 811 and 817 and DSDP Leg 21 Site 209, Proceedings of the Ocean Drilling Program, 133 Scientific Results, https://doi.org/10.2973/ODP.PROC.SR.133.230.1993, 1993.
Isern, A. R., McKenzie, J. A., and Feary, D. A.: The role of sea-surface temperature as a control on carbonate platform development in the western Coral Sea, Palaeogeogr. Palaeocl., 124, 247–272, https://doi.org/10.1016/0031-0182(96)80502-5, 1996.
Isern, A. R., Anselmetti, F. S., and Blum, P.: A Neogene Carbonate Platform, Slope, and Shelf Edifice Shaped by Sea Level and Ocean Currents, Marion Plateau (Northeast Australia), Seismic Imaging of Carbonate Reservoirs and Systems, edited by: Eberli, G. P., Masaferro, J. L., and Sarg, J. F. R., AAPG, https://doi.org/10.1306/M81928, 2004.
John, C. M. and Mutti, M.: Relative Control of Paleoceanography, Climate, and Eustasy over Heterozoan Carbonates: A Perspective from Slope Sediments of the Marion Plateau (ODP LEG 194), J. Sediment. Res., 75, 216–230, https://doi.org/10.2110/jsr.2005.017, 2005.
Jöhnck, J., Kuhnt, W., Holbourn, A., and Andersen, N.: Variability of the Indian Monsoon in the Andaman Sea Across the Miocene-Pliocene Transition, Paleoceanogr. Paleoclimatol., 35, e2020PA003923, https://doi.org/10.1029/2020PA003923, 2020.
Katz, M. E. and Miller, K. G.: Neogene Subsidence along the Northeastern Australian Margin: Benthic Foraminiferal Evidence, Proceedings of the Ocean Drilling Program, 133 Scientific Results, https://doi.org/10.2973/ODP.PROC.SR.133.242.1993, 1993.
Kim, J.-H., van der Meer, J., Schouten, S., Helmke, P., Willmott, V., Sangiorgi, F., Koç, N., Hopmans, E. C., Damsté, J. S. S., and Damste, J. S. S.: New indices and calibrations derived from the distribution of crenarchaeal isoprenoid tetraether lipids: Implications for past sea surface temperature reconstructions, Geochim. Cosmochim. Ac., 74, 4639–4654, https://doi.org/10.1016/j.gca.2010.05.027, 2010a.
Kim, J.-H., der Meer, J., Schouten, S., Helmke, P., Willmott, V., Sangiorgi, F., Koç, N., Hopmans, E. C., and Damsté, S.: New indices and calibrations derived from the distribution of crenarchaeal isoprenoid tetraetherlipids: Implications for past sea surface temperature reconstructions, Geochim. Cosmochim. Ac., 74, 4639–4654, 2010b.
Laugié, M., Michel, J., Pohl, A., Poli, E., and Borgomano, J.: Global distribution of modern shallow-water marine carbonate factories: a spatial model based on environmental parameters, Sci. Rep., 9, 16432, https://doi.org/10.1038/s41598-019-52821-2, 2019.
Liu, X., Huber, M., Foster, G. L., Dessler, A., and Zhang, Y. G.: Persistent high latitude amplification of the Pacific Ocean over the past 10 million years, Nat. Commun., 13, 7310, https://doi.org/10.1038/s41467-022-35011-z, 2022.
Locarnini, R. A., Mishonov, A. V, Baranova, O. K., Boyer, T. P., Zweng, M. M., Garcia, H. E., Reagan, J. R., Seidov, D., Weathers, K. W., Paver, C. R., and Smolyar, I. V: World Ocean Atlas, 1, 52 pp., https://www.ncei.noaa.gov/products/world-ocean-atlas (last access: 1 April 2024), 2019.
Lough, J. M.: Coral calcification from skeletal records revisited, Mar. Ecol. Prog. Ser., 373, 257–264, https://doi.org/10.3354/MEPS07398, 2008.
Lough, J. M. and Barnes, D. J.: Environmental controls on growth of the massive coral Porites, J. Exp. Mar. Biol. Ecol., 245, 225–243, https://doi.org/10.1016/S0022-0981(99)00168-9, 2000.
Lough, J. M. and Cantin, N. E.: Perspectives on massive coral growth rates in a changing ocean, Biol. Bull., 226, 187–202, 2014.
Lübbers, J., Kuhnt, W., Holbourn, A. E., Bolton, C. T., Gray, E., Usui, Y., Kochhann, K. G. D., Beil, S., and Andersen, N.: The Middle to Late Miocene “Carbonate Crash” in the Equatorial Indian Ocean, Paleoceanogr. Paleoclimatol., 34, 813–832, https://doi.org/10.1029/2018PA003482, 2019.
Martin, P. E., Macdonald, F. A., McQuarrie, N., Flowers, R. M., and Maffre, P. J. Y.: The rise of New Guinea and the fall of Neogene global temperatures, P. Natl. Acad. Sci. USA, 120, 40, https://doi.org/10.1073/pnas.2306492120, 2023.
Martinot, C., Bolton, C. T., Sarr, A.-C., Donnadieu, Y., Garcia, M., Gray, E., and Tachikawa, K.: Drivers of Late Miocene Tropical Sea Surface Cooling: A New Perspective From the Equatorial Indian Ocean, Paleoceanogr. Paleoclimatol., 37, e2021PA004407, https://doi.org/10.1029/2021PA004407, 2022.
Mathew, M., Makhankova, A., Menier, D., Sautter, B., Betzler, C., and Pierson, B.: The emergence of Miocene reefs in South China Sea and its resilient adaptability under varying eustatic, climatic and oceanographic conditions, Sci. Rep., 10, 7141, https://doi.org/10.1038/s41598-020-64119-9, 2020.
Miller, K. G., Browning, J. V., John Schmelz, W., Kopp, R. E., Mountain, G. S., and Wright, J. D.: Cenozoic sea-level and cryospheric evolution from deep-sea geochemical and continental margin records, Sci. Adv., 6, eaaz1346 https://doi.org/10.1126/SCIADV.AAZ1346, 2020.
Müller, P. J., Kirst, G., Ruhland, G., von Storch, I., and Rosell-Mele, A.: Calibration of the alkenone paleotemperature index U-37(K′) based on core-tops from the eastern South Atlantic and the global ocean (60° N–60° S), Geochim. Cosmochim. Ac., 62, 1757–1772, 1998.
Nairn, M. G., Lear, C. H., Sosdian, S. M., Bailey, T. R., and Beavington-Penney, S.: Tropical Sea Surface Temperatures Following the Middle Miocene Climate Transition From Laser-Ablation ICP-MS Analysis of Glassy Foraminifera, Paleoceanogr. Paleoclimatol., 36, e2020PA004165, https://doi.org/10.1029/2020PA004165, 2021.
Pelejero, C. and Calvo, E.: The upper end of the U temperature calibration revisited, Geochem. Geophy. Geosy., 4, 1014, https://doi.org/10.1029/2002gc000431, 2003.
Perrin, C. and Kiessling, W.: Latitudinal Trends in Cenozoic Reef Patterns and their Relationship to Climate, in: Carbonate Systems during the Oligocene–Miocene Climatic Transition, Wiley, 17–33, https://doi.org/10.1002/9781118398364.ch2, 2012.
Petrick, B., McClymont, E. L., Littler, K., Rosell-Melé, A., Clarkson, M. O., Maslin, M., Röhl, U., Shevenell, A. E., and Pancost, R. D.: Oceanographic and climatic evolution of the southeastern subtropical Atlantic over the last 3.5 Ma, Earth Planet Sc. Lett., 492, 12–21, https://doi.org/10.1016/j.epsl.2018.03.054, 2018.
Petrick, B., Martínez-García, A., Auer, G., Reuning, L., Auderset, A., Deik, H., Takayanagi, H., De Vleeschouwer, D., Iryu, Y., and Haug, G. H.: Glacial Indonesian Throughflow weakening across the Mid-Pleistocene Climatic Transition, Sci. Rep., 9, 16995, https://doi.org/10.1038/s41598-019-53382-0, 2019.
Petrick, B., Reuning, L., Auer, G., Zhang, Y., Pfeiffer, M., and Schwark, L.: Warm, not cold temperatures contributed to a Late Miocene reef decline in the Coral Sea, Sci. Rep., 13, 4015, https://doi.org/10.1038/s41598-023-31034-8, 2023.
Petrick, B., Reuning, L., Pfeiffer, M., Auer, G., and Schwark, L.: ODP site 811 SSTs, Zenodo [data set], https://doi.org/10.5281/zenodo.10902264, 2024.
Rattanasriampaipong, R., Zhang, Y. G., Pearson, A., Hedlund, B. P., and Zhang, S.: Archaeal lipids trace ecology and evolution of marine ammonia-oxidizing archaea, P. Natl. Acad. Sci. USA, 119, e2123193119, https://doi.org/10.1073/PNAS.2123193119, 2022.
Renema, W., Bellwood, D. R., Braga, J. C., Bromfield, K., Hall, R., Johnson, K. G., Lunt, P., Meyer, C. P., McMonagle, L. B., Morley, R. J., O'Dea, A., Todd, J. A., Wesselingh, F. P., Wilson, M. E. J., and Pandolfi, J. M.: Hopping hotspots: global shifts in marine biodiversity, Science, 321, 654–657, https://doi.org/10.1126/science.1155674, 2008.
Rosleff-Soerensen, B., Reuning, L., Back, S., and Kukla, P.: Seismic geomorphology and growth architecture of a Miocene barrier reef, Browse Basin, NW-Australia, Mar. Petrol. Geol., 29, 233–254, https://doi.org/10.1016/j.marpetgeo.2010.11.001, 2012.
Rosleff-Soerensen, B., Reuning, L., Back, S., and Kukla, P. A.: The response of a basin-scale Miocene barrier reef system to long-term, strong subsidence on a passive continental margin, Barcoo Sub-basin, Australian North West Shelf, Basin Res., 28, 103–123, https://doi.org/10.1111/bre.12100, 2016.
Santodomingo, N., Renema, W., and Johnson, K. G.: Understanding the murky history of the Coral Triangle: Miocene corals and reef habitats in East Kalimantan (Indonesia), Coral Reefs, 35, 765–781, https://doi.org/10.1007/S00338-016-1427-Y, 2016.
Schlitzer, R.: Ocean Data View, https://odv.awi.de (last access: 1 April 2024), 2021.
Schouten, S., Hopmans, E. C., Schefuss, E., and Sinninghe Damsté, J. S.: Distributional variations in marine crenarchaeotal membrane lipids: a new tool for reconstructing ancient sea water temperatures?, Earth Planet Sc. Lett., 204, 265–274, 2002.
Schouten, S., Hopmans, E. C., Rosell-Melé, A., Pearson, A., Adam, P., Bauersachs, T., Bard, E., Bernasconi, S. M., Bianchi, T. S., Brocks, J. J., Carlson, L. T., Castañeda, I. S., Derenne, S., Selver, A. D., Dutta, K., Eglinton, T., Fosse, C., Galy, V., Grice, K., Hinrichs, K.-U., Huang, Y., Huguet, A., Huguet, C., Hurley, S., Ingalls, A., Jia, G., Keely, B., Knappy, C., Kondo, M., Krishnan, S., Lincoln, S., Lipp, J., Mangelsdorf, K., Martínez-García, A., Ménot, G., Mets, A., Mollenhauer, G., Ohkouchi, N., Ossebaar, J., Pagani, M., Pancost, R. D., Pearson, E. J., Peterse, F., Reichart, G.-J., Schaeffer, P., Schmitt, G., Schwark, L., Shah, S. R., Smith, R. W., Smittenberg, R. H., Summons, R. E., Takano, Y., Talbot, H. M., Taylor, K. W. R., Tarozo, R., Uchida, M., van Dongen, B. E., Van Mooy, B. A. S., Wang, J., Warren, C., Weijers, J. W. H., Werne, J. P., Woltering, M., Xie, S., Yamamoto, M., Yang, H., Zhang, C. L., Zhang, Y., Zhao, M., and Damsté, J. S. S.: An interlaboratory study of TEX86 and BIT analysis of sediments, extracts, and standard mixtures, Geochem. Geophy. Geosy. 14, 5263–5285, https://doi.org/10.1002/2013GC004904, 2013.
Shipboard Scientific Party: Principal Results and Summary, in: Proceedings of the Ocean Drilling Program, 133 Initial Reports, vol. 133, edited by: Davies, P. J., McKenzie, J. A., and Palmer-Julson, A., Ocean Drilling Program, 59–72, https://doi.org/10.2973/odp.proc.ir.133.103.1991, 1991.
Sinninghe Damsté, J. S., Ossebaar, J., Schouten, S., and Verschuren, D.: Distribution of tetraether lipids in the 25-ka sedimentary record of Lake Challa: extracting reliable TEX86 and MBT/CBT palaeotemperatures from an equatorial African lake, Quaternary Sci. Rev., 50, 43–54, https://doi.org/10.1016/j.quascirev.2012.07.001, 2012.
Spalding, M. D., Fox, H. E., Allen, G. R., Davidson, N., Ferdaña, Z. A., Finlayson, M., Halpern, B. S., Jorge, M. A., Lombana, A., Lourie, S. A., Martin, K. D., McManus, E., Molnar, J., Recchia, C. A., and Robertson, J.: Marine Ecoregions of the World: A Bioregionalization of Coastal and Shelf Areas, Bioscience, 57, 573–583, https://doi.org/10.1641/B570707, 2007.
Steinthorsdottir, M., Coxall, H. K., de Boer, A. M., Huber, M., Barbolini, N., Bradshaw, C. D., Burls, N. J., Feakins, S. J., Gasson, E., Henderiks, J., Holbourn, A. E., Kiel, S., Kohn, M. J., Knorr, G., Kürschner, W. M., Lear, C. H., Liebrand, D., Lunt, D. J., Mörs, T., Pearson, P. N., Pound, M. J., Stoll, H., and Strömberg, C. A. E.: The Miocene: The Future of the Past, Paleoceanogr. Paleoclimatol., 36, e2020PA004037, https://doi.org/10.1029/2020PA004037, 2021.
Strömberg, C. A. E. and Strömberg, S.: Evolution of Grasses and Grassland Ecosystems, Annu. Rev. Earth Pl. Sc. 39, 517–544, https://doi.org/10.1146/annurev-earth-040809-152402, 2011.
Tagliaro, G., Fulthorpe, C. S., Gallagher, S. J., McHugh, C. M., Kominz, M., and Lavier, L. L.: Neogene siliciclastic deposition and climate variability on a carbonate margin: Australian Northwest Shelf, Mar. Geol., 403, 285–300, https://doi.org/10.1016/J.MARGEO.2018.06.007, 2018.
Tanner, T., Hernández-Almeida, I., Drury, A. J., Guitián, J., and Stoll, H.: Decreasing Atmospheric CO2 During the Late Miocene Cooling, Paleoceanogr. Paleoclimatol., 35, e2020PA003925, https://doi.org/10.1029/2020PA003925, 2020.
Taylor, K. W. R., Huber, M., Hollis, C. J., Hernandez-Sanchez, M. T., and Pancost, R. D.: Re-evaluating modern and Palaeogene GDGT distributions: Implications for SST reconstructions, Global Planet. Change, 108, 158–174, https://doi.org/10.1016/j.gloplacha.2013.06.011, 2013.
Thronberens, S., Back, S., Bourget, J., Allan, T., and Reuning, L.: 3-D seismic chronostratigraphy of reefs and drifts in the Browse Basin, NW Australia, GSA Bulletin, 134, 3155–3175, https://doi.org/10.1130/B36286.1, 2022.
Van Hinsbergen, D. J. J., De Groot, L. V., Van Schaik, S. J., Spakman, W., Bijl, P. K., Sluijs, A., Langereis, C. G., and Brinkhuis, H.: A Paleolatitude Calculator for Paleoclimate Studies, PLoS One, 10, e0126946, https://doi.org/10.1371/JOURNAL.PONE.0126946, 2015.
Weijers, J. W. H., Schouten, S., Hopmans, E. C., Geenevasen, J. A. J., David, O. R. P., Coleman, J. M., Pancost, R. D., and Sinninghe Damsté, J. S.: Membrane lipids of mesophilic anaerobic bacteria thriving in peats have typical archaeal traits, Environ. Microbiol., 8, 648–657, https://doi.org/10.1111/j.1462-2920.2005.00941.x, 2006.
Wen, Y., Zhang, L., Holbourn, A. E., Zhu, C., Huntington, K. W., Jin, T., Li, Y., and Wang, C.: CO2-forced Late Miocene cooling and ecosystem re-organizations in East Asia, P. Natl. Acad. Sci. USA, 120, e2214655120, https://doi.org/10.1073/pnas.2214655120, 2023.
Westerhold, T., Marwan, N., Drury, A. J., Liebrand, D., Agnini, C., Anagnostou, E., Barnet, J. S. K., Bohaty, S. M., De Vleeschouwer, D., Florindo, F., Frederichs, T., Hodell, D. A., Holbourn, A. E., Kroon, D., Lauretano, V., Littler, K., Lourens, L. J., Lyle, M., Pälike, H., Röhl, U., Tian, J., Wilkens, R. H., Wilson, P. A., and Zachos, J. C.: An astronomically dated record of Earth's climate and its predictability over the last 66 million years, Science, 369, 1383–1387, https://doi.org/10.1126/science.aba6853, 2020.
Wilson, P. A., Norris, R. D., and Cooper, M. J.: Testing the Cretaceous greenhouse hypothesis using glassy foraminiferal calcite from the core of the Turonian tropics on Demerara Rise, Geology, 30, 607–610, https://doi.org/10.1130/0091-7613(2002)030<0607:TTCGHU>2.0.CO;2, 2002.
Zachos, J. C., Stott, L. D., and Lohmann, K. C.: Evolution of early Cenozoic marine temperatures, Paleoceanography, 9, 353–387, https://doi.org/10.1029/93PA03266, 1994.
Zhang, Y. G., Zhang, C. L., Liu, X.-L., Li, L., Hinrichs, K.-U., and Noakes, J. E.: Methane Index: A tetraether archaeal lipid biomarker indicator for detecting the instability of marine gas hydrates, Earth Planet. Sc. Lett., 307, 525–534, https://doi.org/10.1016/J.EPSL.2011.05.031, 2011.
Zhang, Y. G., Pagani, M., and Liu, Z.: A 12-Million-Year Temperature History of the Tropical Pacific Ocean, Science, 344, 84–87, https://doi.org/10.1126/science.1246172, 2014.
Zhang, Y. G., Pagani, M., and Wang, Z.: Ring Index: A new strategy to evaluate the integrity of TEX86 paleothermometry, Paleoceanography, 31, 220–232, https://doi.org/10.1002/2015PA002848, 2016.