the Creative Commons Attribution 4.0 License.
the Creative Commons Attribution 4.0 License.
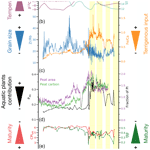
Deglacial export of pre-aged terrigenous carbon to the Bay of Biscay
Wanyee Wong
Jens Hefter
Hendrik Grotheer
Tommaso Tesi
Torben Gentz
Karin Zonneveld
Gesine Mollenhauer
The last deglaciation is the most recent relatively well-documented period of pronounced and fast climate warming, and, as such, it holds important information for our understanding of the climate system. Notably, while research into terrestrial organic carbon reservoirs has been instrumental in exploring the possible sources of atmospheric carbon dioxide during periods of rapid change, the underlying mechanisms are not fully understood. Here we investigate the mobilization of organic matter to the Bay of Biscay, located in the north-eastern Atlantic Ocean off the coasts of France and Spain. Specifically, we focus on the area that was the mouth of the Channel River during the last deglaciation, where an enhanced terrigenous input has been reported for the last glacial–interglacial transition. We conducted a comprehensive suite of biomarker analyses (e.g. n-alkanes, hopanes and n-alkanoic acids) and isotopic investigations (radiocarbon dating and δ13C measurements) on a high-resolution sedimentary archive. The present study provides the first direct evidence for the fluvial supply of immature and ancient terrestrial organic matter to the core location. Moreover, our results reveal the possibility of permafrost carbon export to the ocean, driven by processes such as deglacial warming and glacial erosion. These findings are consistent with observations from other regions characterized by present or past permafrost conditions on land, which have shown that permafrost thaw and glacial erosion can lead to carbon remobilization, potentially influencing atmospheric carbon dioxide levels.
- Article
(2814 KB) - Full-text XML
-
Supplement
(5898 KB) - BibTeX
- EndNote
High-latitude permafrost soils hold ca. 1000 Pg of soil organic carbon (C) in the upper 3 m of the northern circumpolar region (Hugelius et al., 2014). This immense amount of C is in the form of frozen organic matter (OM), the thawing of which releases greenhouse gases, inducing positive feedback mechanisms that have implications for the C cycle on a global scale (Zimov et al., 2006; Schuur et al., 2008, 2009; Hugelius et al., 2014; Schuur et al., 2015). While current climate change raises concerns about the stability of these massive pools of organic C (Vonk et al., 2012; Schneider Von Deimling et al., 2015), the dynamic character of Earth’s climate means that past trends and variability can be examined to improve future projections of this effect. The hypothesis of a combined contribution of ancient terrestrial C, potentially derived from thawing permafrost, and marine C sources to elevated atmospheric levels of carbon dioxide (CO2) and methane (CH4) during the last deglaciation is discussed in several studies (Ciais et al., 2012; Köhler et al., 2014; Bauska et al., 2016; Crichton et al., 2016; Simmons et al., 2016). Over the course of the Last Glacial Maximum (LGM), large expanses of continuous permafrost were found in the Eurasian continent, covering much of central and western Europe, in areas where permafrost cover today no longer exists (Vandenberghe and Pissart, 1993; Levavasseur et al., 2011; Vandenberghe et al., 2012; Žák et al., 2012; Schaefer et al., 2014; Vandenberghe et al., 2014) (Fig. 1). These regions comprise the southern edge of the LGM permafrost area and, according to Köhler et al. (2014), are likely to have experienced a rapid loss of massive amounts of ancient C as a result of thawing during the last deglaciation. However, while direct evidence for the deglacial remobilization of ancient C from permafrost has been reported for the Arctic (Tesi et al., 2016; Keskitalo et al., 2017; Martens et al., 2019, 2020; Wu et al., 2022) and subarctic (Winterfeld et al., 2018; Meyer et al., 2019), similar data are still lacking for the European realm, where the phenomenon has been suggested on the basis of enhanced terrigenous biomarker concentrations in sediment cores (Ménot et al., 2006; Rostek and Bard, 2013; Soulet et al., 2013).
During the LGM, continental glaciers were part of the European landscape. The Fennoscandian (FIS) and the British–Irish (BIIS) ice sheets covered most of Britain, Ireland, northern Europe and the North Sea (Bowen et al., 2002; Svendsen et al., 2004; Mangerud et al., 2004), contributing to the lower eustatic sea level and altering coastlines (e.g. Fairbanks, 1989; Lambeck, 1997; Lambeck et al., 2014). This sea-level lowstand, paired with the configuration of the BIIS and the FIS, led to a reorganization of major European drainage basins, with continental runoff being funnelled through the English Channel (Gibbard, 1988). The so-called Fleuve Manche or Channel River received the runoff of major European rivers, carrying meltwaters from glaciers and ice sheets (e.g. Antoine et al., 2003; Bourillet et al., 2003) (Fig. 1). As a consequence, changes in the hydrological cycle in Europe during the last glacial–interglacial transition induced a strong response from this system, resulting in increased water flow in the Channel River and its tributaries (e.g. Ménot et al., 2006; Toucanne et al., 2009, 2010). Permafrost developed in the glacier-free areas of the continent, extending across a large portion of the Channel River (Fig. 1), and towards the end of the last glaciation it is likely to have reached its maximum extent, with discontinuous permafrost present in regions almost as far south as the Mediterranean Sea (Vandenberghe et al., 2014).
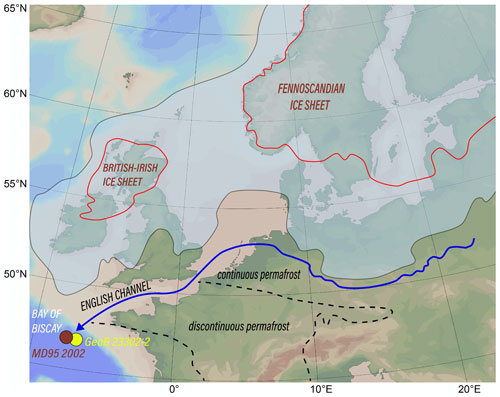
Figure 1North-western Europe during the LGM. The blue arrow indicates the downstream course of the Channel River from the eastern flank of the FIS. The dashed lines show the distribution of permafrost (Renssen and Vandenberghe, 2003, and references therein), and the red contours indicate the approximate limits of the ice sheets at ca. 17 ka (Patton et al., 2017). The yellow dot illustrates the location where core GeoB23302-2, used in the present study, was retrieved. Map based on that in Ménot et al. (2006), who studied core MD95 2002 from a nearby location (red dot).
Permafrost encompasses a diverse range of organic-rich deposits, including ancient peat, organic-rich soils and potentially mineral soils, all of which can become stabilized (frozen) under permafrost conditions, preserving C within them. There is evidence for the presence of several peatlands with active peat deposition in northern latitudes (> 40∘ N), including northern Europe, during the last interglacial (130–116 kyr BP; Treat et al., 2019). Although the occurrence of permafrost during the LGM likely resulted in the long-term burial of peatland OM, deglacial permafrost thawing may have led to the fluvial export of peat-derived OM to the ocean (see e.g. Schefuß et al., 2016; Garcin et al., 2022). In addition to permafrost, petrogenic material carried by glacial meltwater may have been another source of fossil OM to the oceans during the last deglaciation. Following glacial retreat, the mechanical erosion of bedrock such as oil shales mobilizes petrogenic C, which is transported as finely ground glacial meal to the oceans (Koppes and Hallet, 2002, 2006). Therefore, if we are to accurately quantify the impact of the permafrost C feedback on Earth's climate, it is imperative to distinguish between the possible origins of the OM deposited on the continental margins over the course of the last deglaciation. Considering the presence of shale formations in Europe (e.g. Zhao et al., 2022), our study explores two primary hypotheses regarding the origin of OM: permafrost-derived OM, which includes peat preserved in past permafrost-covered European regions, and OM originating from petrogenic sources. Here, we analysed organic biomarkers and conducted compound-specific radiocarbon (14C) measurements on n-alkanoic acids isolated from a high-resolution, well-dated marine sediment core retrieved from the Channel River outflow to evaluate these hypotheses.
Herein, we provide an overview of our analytical approach; for in-depth descriptions of each method and the specific details of our analyses, please refer to the following subsections. All the elemental, isotopic and biomarker analyses described in this section were conducted as part of the present study. In this research we used several analytical tools to examine core GeoB23302-2 (47∘26.61′ N, 8∘28.67′ W; 2167 m water depth) (Fig. 1). The chronology of the sedimentary sequence was established using an age–depth model constructed with the 14C ages of planktic foraminifera with the OxCal software (Bronk Ramsey, 1995, 2009a). Elemental ratios were obtained through X-ray fluorescence (XRF) core scanning. The coarse fraction of sediments tends to be enriched in zirconium (Zr), while rubidium (Rb) is found in fine-grained minerals. Therefore, here we report the ratio Zr Rb as an elemental measure of grain size, which has been used as a proxy for river runoff (Dypvik and Harris, 2001; Kylander et al., 2011; Wang et al., 2011; Wu et al., 2020). Similarly, given that iron (Fe) is normally associated with continental weathering products and that the calcium (Ca) content in the sediment primarily reflects the presence of marine carbonate, here we use the Fe Ca ratio as a provenance indicator, reflecting variations in terrigenous sediment delivery (Arz et al., 1999; Itambi et al., 2009; Dickson et al., 2010; Perez et al., 2016). We analysed lipid biomarkers using solvent extraction and gas chromatography and calculated n-alkane-derived indices, namely the carbon-number preference index (CPIalk) (e.g. Bray and Evans, 1961; Marzi et al., 1993) and the proxy ratio Paq (Ficken et al., 2000), which are commonly used in environmental investigations (e.g. Nichols et al., 2006; Rommerskirchen et al., 2006; Zhou et al., 2012; He et al., 2020; Feurdean et al., 2021) to assess the degree of OM degradation and reconstruct the temporal evolution of continental vegetation systems, respectively. The CPIalk is based on the ratio of odd to even n-alkanes, providing information about the distribution of these compounds in the samples, while the Paq reflects the predominance of long-chain n-alkanes in terrestrial vascular plants as opposed to algae and macrophytes, which primarily synthesize short- to mid-chain n-alkanes (Bianchi and Canuel, 2011). The Paq ratio indicates the relative input of aquatic macrophytes and terrestrial plants to the sediment (Ficken et al., 2000). The prevalence of odd-numbered n-alkanes in fresh material implies that the CPIalk can serve as an indicator of OM degradation (Bray and Evans, 1961; Marzi et al., 1993; Meyers and Ishiwatari, 1993). To further assess the presence of petrogenic OM, we used the fractional abundance of hopanes of biological origin, e.g. bacteria-derived hopanes, in relation to their diagenetic isomers (fββ) (Meyer et al., 2019). Here, the branched and isoprenoid tetraether (BIT) index (Hopmans et al., 2004), based on the relative abundance of branched glycerol dialkyl glycerol tetraether lipids (GDGTs) characteristic of terrestrial bacteria and the isoprenoid GDGT crenarchaeol produced by marine Thaumarchaeota, is used as a proxy for the input of terrestrially sourced OM. Carbon isotope analysis (δ13C) of bulk samples was conducted as part of our investigation into their origin. Finally, given that the results of bulk 14C dating reflect the 14C content of a heterogeneous mixture of compounds possibly derived from distinct sources, here we further address the provenance of the OM by using compound-specific 14C analyses of high-molecular-weight n-alkanoic acids (C26:0, C28:0 and C30:0) – which are typically derived from vascular plants (Bianchi and Canuel, 2011) – from specific depths in the core. This approach has been successfully employed for the identification of ancient terrigenous material export at other sites (e.g. Winterfeld et al., 2018; Meyer et al., 2019; Wu et al., 2022).
2.1 Sampling and core chronology
Core GeoB23302-2 was recovered from the Celtic Margin, off the English Channel (47∘26.61′ N, 8∘28.67′ W; 2167 m water depth) (Fig. 1), with the help of a gravity corer during cruise MSM 79 of the research vessel Maria S. Merian. The core location is in close proximity to the site where core MD95 2002, which has been studied in previous publications (e.g. Ménot et al., 2006; Toucanne et al., 2015), was retrieved (47∘27′ N, 8∘32′ W) (see Fig. 1). The chronology of our 700 cm core was established based on seven radiocarbon accelerator mass spectrometry (14C-AMS) measurements of planktic foraminifera (G. bulloides and N. pachyderma) picked at specific depths (Table 1). The preparation and measurement of these samples followed well-established protocols routinely run at the MICADAS 14C laboratory of the Alfred Wegener Institute (AWI) (Mollenhauer et al., 2021). The 14C ages were uploaded to the OxCal software version 4.4.2 (Bronk Ramsey, 1995, 2009a), and assuming that the deposition is a Poisson process, the P Sequence model was employed for the construction of an age–depth model for core GeoB23302-2 (Bronk Ramsey, 2008; Bronk Ramsey and Lee, 2013). This deposition model (Fig. S2 in the Supplement) uses the global marine calibration curve Marine20 (Heaton et al., 2020) and a local marine reservoir correction ΔR of 94 ± 45 14C yr (Tisnérat-Laborde et al., 2010). It is important to note that while Marine20 incorporates larger marine reservoir age estimates for the last glacial period than Marine13, these estimates are considered more realistic due to methodological improvements in the former (Heaton et al., 2020, 2023). A general outlier analysis was employed to account for possible outliers within the chronological model (Bronk Ramsey, 2009b). The code is available in the Supplement accompanying this paper.
2.2 Elemental analyses
The XRF characterization of core GeoB23302-2 was performed using the XRF Core Scanner II (AVAATECH serial no. 2) at the Center for Marine Environmental Sciences (MARUM), University of Bremen, Germany. Measurements were performed at 1 cm intervals for the upper 3.5 m of the core and at every 2 cm for the remaining section. The scan resolution was set to 1 cm with two running rounds, during which the elements were detected with 10 and 30 kV of tube voltage. In order to account for the closed sum effects of water content, grain size and OM amount (e.g. Weltje and Tjallingii, 2008), we report the elemental ratios Zr Rb and Fe Ca.
2.3 Biomarker analyses and derived indices
Sediment samples taken at 10 cm intervals from core GeoB23302-2 were freeze-dried and homogenized. For each depth, approximately 3 g of sediment was subsampled and underwent ultrasonic extraction with a mixture of dichloromethane : methanol 9:1 (v:v). This step was repeated three times, and the total lipid extracts obtained were then saponified with 0.1 M potassium hydroxide (KOH) in methanol : water 9:1 at 80 ∘C for 2 h. This procedure resulted in the separation of the neutral lipids and n-alkanoic acids fractions, which were subsequently extracted using n-hexane and dichloromethane (at pH 1), respectively. Next, silica gel chromatography was employed to further split the neutral lipids via elution with n-hexane and dichloromethane : methanol 1:1 (v:v), yielding the n-alkane and GDGT subfractions, respectively. The n-alkane concentrations were measured via gas chromatography (GC) using a 7890A GC (Agilent Technologies) equipped with a flame ionization detector (FID) and DB-5MS fused silica capillary columns (60 m, ID 250 µm, 0.25 µm film coupled to a 5 m, ID 530 µm deactivated fused silica precolumn). Retention times and the comparison with an n-alkane standard were used for the identification of different compounds, whereas quantifications were achieved through the use of an internal standard (squalane) added to the sample prior to extraction. We calculated n-alkane-derived indices, namely the CPIalk (e.g. Bray and Evans, 1961; Marzi et al., 1993),
and the Paq (Ficken et al., 2000),
Hopanes were analysed via GC coupled with time-of-flight mass spectrometry (GC-TOF-MS), and such a system consisted of a LECO Pegasus III (LECO Corp., St. Joseph, MI) interfaced to an Agilent 6890 GC, which was equipped with a temperature-programmable cooled injection system (CIS4, Gerstel). The measurements were performed using the instrumental set-up described in Hefter (2008), and identification was achieved through the relative retention times and mass spectra. The sum of 191 and 205 was used for the quantification of homohopane isomers (C31), namely the 17β,21β (H), 22R homohopane; the 17β,21α (H), 22R + 17β,21α (H), 22S homohopanes; the 17α,21β (H), 22R homohopane; and the 17α,21β (H), 22S homohopane. Next, the fββ was calculated (Meyer et al., 2019):
The analyses of branched and isoprenoid GDGTs by high-performance liquid chromatography (HPLC) were performed on an Agilent 1200 series HPLC system coupled to an Agilent 6120 single-quadrupole MS via an atmospheric pressure chemical ionization interface (APCI), broadly following the method described in Hopmans et al. (2016). The chromatographic separation of individual GDGTs was achieved via the use of two ultra-performance liquid chromatography (UPLC) silica columns in series (Waters Acquity BEH HILIC, 2.1 mm × 150 mm, 1.7 µm and a 2.1 mm × 5 mm pre-column of the same material) maintained at 30 ∘C. Positive-ion APCI-MS and selective ion monitoring (SIM) of (M + H)+ ions (Sinninghe Damsté et al., 2000) or ion-source fragmentation products of OH-GDGTs (Liu et al., 2012) allowed the identification of GDGTs. Quantification was performed with the use of an internal standard (C46-GDGT) added prior to extraction. For this research, we calculated the BIT index (Hopmans et al., 2004):
where the roman numerals refer to specific GDGTs characteristic of terrestrial bacteria, and cren stands for crenarchaeol, which is derived from marine planktonic Thaumarchaeota.
2.4 Compound-specific radiocarbon analyses (CSRA)
Soxhlet extraction was employed for the compound-specific 14C dating of high-molecular-weight n-alkanoic acids. For that purpose, approximately 100 g of freeze-dried and homogenized sediment taken from selected depths in core GeoB23302-2 was extracted for 48 h using a mixture of dichloromethane : methanol 9:1 (v:v). Total lipid extracts were saponified with 0.1 M KOH in methanol : water 9:1 at 80 ∘C for 2 h, and the n-alkanoic acids were recovered from the saponified solution using n-hexane at pH 1. Next, n-alkanoic acids were methylated at 80 ∘C overnight in a nitrogen atmosphere with HCl and methanol of known 14C signature to yield the fatty acid methyl esters (FAMEs) that were later extracted with n-hexane. Silica gel chromatography was employed to separate FAMEs from polar compounds. The n-C26:0, n-C28:0 and n-C30:0 alkanoic acids underwent purification via preparative capillary GC (PC-GC; Eglinton et al., 1996) on an Agilent HP6890N GC with a Gerstel cooled injection system (CIS) connected to a Gerstel preparative fraction collector (Kusch et al., 2010). A Restek Rxi-1ms fused silica capillary column (30 m, 0.53 mm diameter, 1.5 µm film thickness) equipped the GC. Injection was performed stepwise with 5 µL per injection, and, at the end of the process, the purity of the FAMEs was checked by analysing aliquots of the samples via GC-FID. The purified FAMEs were transferred to tin capsules (25 µL volume, ELEMENTAR) using dichloromethane, dried on a hot plate at 40 ∘C and packed. An Elementar vario ISOTOPE EA (elemental analyser) was used for the combustion of the samples, generating CO2 with carbon isotopic ratios directly determined by the connected MICADAS system. Reference standards (oxalic acid II, SRM 4990C) and 14C-free CO2 gas had their 14C content measured together with the samples. The BATS software (Wacker et al., 2010) was used for blank corrections and standard normalization, and the final results are reported as fraction modern carbon (Fm).
2.5 Assessment and correction of CSRA procedure blank
The preparation procedures for CSRA introduce exogenous C, i.e. contaminants, to samples. The degree of contamination varies according to the methods employed, and, in our case, processes such as column bleed and carryover during prep-GC compound isolation may contribute to this. For this reason, assessing the Fm and the size of the blank (Fmblank and mblank, respectively) is essential for accurate results. Here, in-house reference samples of 14C-free Messel shale (Fm=0) and modern apple peel () underwent the same pre-treatment as samples of unknown age, and their results were used for blank correction following the method outlined in Sun et al. (2020). Isotopic mass balance was employed in order to make a correction for the methyl group added during the derivatization of the samples. Uncertainties were fully propagated.
2.6 Pre-depositional 14C ages of terrigenous compounds
The Δ14C values of the n-alkanoic acids analysed here were corrected for radioactive decay between 1950 and 2021, which is the year of measurement. These values were then used to calculate the Δ14C values at the time of deposition:
where λ is a decay constant ( yr−1), and t is the time of deposition. The Δ14C values of the atmosphere contemporaneous with the compounds (Δ14Catm) were obtained from comparison with the IntCal20 dataset (Reimer et al., 2020) using the age ranges given by the deposition model for the respective sediment layers. Finally, pre-depositional 14C ages for the n-alkanoic acids were given by
These calculations follow the method outlined in Schefuß et al. (2016) and later in Winterfeld et al. (2018), where more details can be found.
2.7 Stable isotope analyses
Carbon stable isotope (δ13C) analyses were carried out on acidified samples (Ag capsules, HCl, 1.5 M) in order to remove the inorganic C (Nieuwenhuize et al., 1994). Analyses were performed using a Thermo Scientific DELTA Q isotope ratio mass spectrometer coupled to a Thermo Scientific FLASH 2000 CHNS/O analyser via Conflo III at the Stable Isotope Laboratory of ISP-CNR. δ13C data are expressed in the conventional delta notation (‰). Isotopic data were calibrated using the IAEA reference material IAEA-CH7 polyethylene (−32.15 ‰ vs. Vienna Pee Dee Belemnite (VPDB)). Throughout the runs, we used other standards with a sediment matrix routinely used in the laboratory to check the reproducibility of measurements. The standard deviation for δ13C measurements was lower than ±0.1‰ based on replicates of sediment standards.
The GeoB23302-2 sediment core spans a period from approximately 25 to 4 cal kyr BP (Table 1). The age–depth model for this core shows a period of enhanced deposition between approximately 20 and 15 cal kyr BP (Fig. S2). An outlier analysis shows that the model represents the foraminifera 14C ages well, with an OxCal overall agreement index of 99 %. From approximately 20.6 until 15 cal kyr BP, the Zr Rb ratio shows a long-term increase, whereas a period of relatively high Fe Ca values is observed at ca. 21–16.4 cal kyr BP (Fig. 2c). Values for the proxy Paq are relatively low during the LGM and show a pronounced increase at approximately 21 cal kyr BP, with higher values towards the late glacial and a sudden decrease at the onset of Heinrich event 1 (H1; iceberg discharge from the Laurentide ice sheet into the North Atlantic Ocean, ca. 17.2 cal kyr BP) (Fig. 2d). This is followed by a sharp increase at approximately 16.5 cal kyr BP and a drop to Holocene values around 16.3 cal kyr BP. The CPIalk and the fββ proxy show relatively low values in the late glacial/early deglaciation when compared to the Holocene (Fig. 2e). During the LGM, the CPIalk values are broadly constant, while an increase is observed at the beginning of H1 followed by a sharp drop around 16.5 cal kyr BP and a return to higher values at approximately 16.2 cal kyr BP. The fββ record shows fluctuations during the LGM, followed by a gradual decrease starting at approximately 18 cal kyr BP and a sharp drop around 16.5 cal kyr BP before returning to higher values at approximately 16.2 cal kyr BP (Fig. 2e). The 14C ages of the long-chain n-alkanoic acids varied from approximately 10 to 39 14C kyr. When converted to pre-depositional age estimates, it is possible to observe that at the peak of our BIT record, around 18 cal kyr BP, compounds pre-aged by up to ca. 25 000 14C yr were delivered to the continental slope (Fig. 2f). Pre-depositional ages broadly follow the BIT record, with younger compounds observed from the end of the BIT peak (ca. 16 cal kyr BP) towards the Holocene. The results of our bulk δ13C analyses corroborate the BIT index record, showing that terrestrial C dominantly contributed to samples during the period of enhanced terrigenous deposition, while the OM in Holocene samples is mostly marine (Table 2).
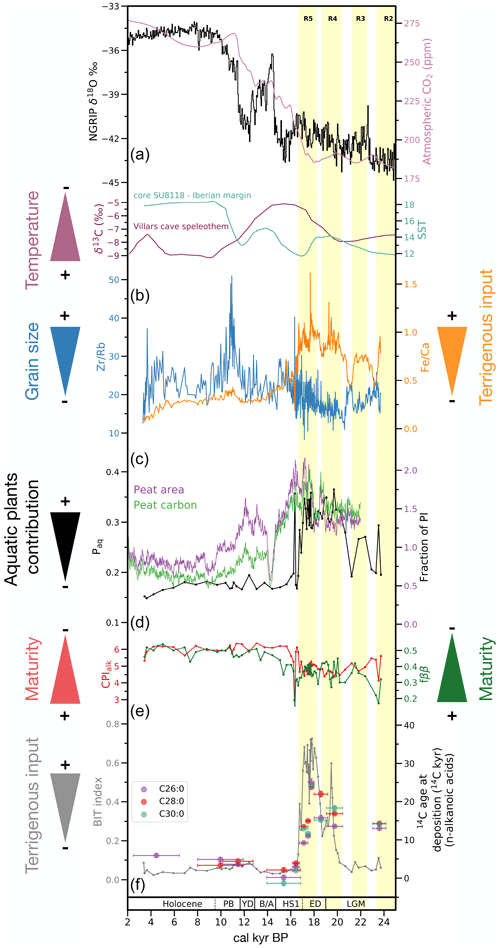
Figure 2(a) NGRIP δ18O (Andersen et al., 2004) and atmospheric CO2 (Köhler et al., 2017) records. (b) Sea surface temperatures in the north-eastern Atlantic Ocean (Bard et al., 2000) and δ13C values from speleothems in western Europe (Wainer et al., 2011). The data were smoothed to eliminate very high-frequency components. (c) Zr Rb and Fe Ca elemental ratios from XRF data. (d) Peatland area (purple) and carbon (green) in Europe as a fraction of pre-industrial (PI) values (0.231 M km2 and 19.6 GtC) (Müller and Joos, 2020) and the Paq index record. (e) fββ and CPIalk records. (f) Pre-depositional 14C ages of n-alkanoic acids from core GeoB23302-2 and BIT index record. Yellow bands mark major flooding events of the Channel River (Toucanne et al., 2015).
4.1 Source of the OM in the sedimentary record
Our results corroborate the previous findings of Ménot et al. (2006), showing a notable increase in the influx of terrigenous OM in the Bay of Biscay during the last deglacial period. Between ca. 20.6 and 15 cal kyr BP, the values of the Zr Rb ratio reflect the deposition of coarse-grained sediments at the core location, which may be associated with enhanced fluvial activity (Wang et al., 2011). A period of relatively high Fe Ca values (ca. 21–16.4 cal kyr BP) is indicative of a greater influx of sediment from land (Fig. 2c). This is consistent with a period of elevated terrestrial contribution to the bulk OM present in the sediment, from approximately 20.5 to 16.5 cal kyr BP, except for a sudden decrease at approximately 19 cal kyr BP, as revealed by the BIT index (see e.g. Hopmans et al., 2004; Herfort et al., 2006; Kim et al., 2006; Schouten et al., 2013; Grotheer et al., 2020) (Fig. 2f). These Fe Ca and BIT patterns are also recorded in core MD95 2002 (see Figs. S3 and S4; Toucanne et al., 2015; Ménot et al., 2006) and a similar pattern of marked Fe Ca peaks, sometimes associated with peaks in OM content, during Heinrich events and much lower values throughout the Holocene has been observed at other sites (Jennerjahn et al., 2004; Lebreiro et al., 2009; Zhang et al., 2015; Crivellari et al., 2018).
Beyond identifying the presence of terrestrial OM transported to the Bay of Biscay via the Channel River during the LGM–Holocene transition, the comprehensive analysis of elemental, geochemical, and isotopic proxies presented here provides insights into the potential sources of this terrigenous OM. Values for the Paq proxy point to a major contribution of OM from aquatic plants between approximately 20.2 and 17.2 cal kyr BP, suggesting the presence of OM sourced from wetland vegetation (Fig. 2d). Our CPIalk record reflects the degree of degradation the sedimentary OM has undergone in its previous terrestrial reservoir or during transportation (cf. Bröder et al., 2018). During the peak of terrigenous deposition, the signal of more mature OM fluvially transported to the continental slope is detected in our CPIalk and fββ records, which reach relatively low values when compared to the Holocene (Fig. 2e). The presence of petrogenic, i.e. thermally mature, material (Farrington and Tripp, 1977; Jeng, 2006) is another factor to consider when interpreting these records. Nonetheless, throughout the archive, the CPIalk values remain above the diagnostic value of petrogenic material (ca. 1) reported by Bray and Evans (1961). Additionally, in contrast with the results of Meyer et al. (2019) for the Bering Sea, in the present study the fββ proxy is not indicative of petrogenic material in the sediment (see the Supplement). This is because the values do not decrease in response to diagenetic transformations but rather due to enhanced inputs of the C31αβR hopane, which is abundant in peat (Inglis et al., 2018).
The compound-specific 14C results disclose that the analysed terrigenous biomarkers are ancient and pre-aged, indicating that they are not contemporaneous with sediment deposition but rather older (Fig. 2f). Despite the large variability in OM residence time in permafrost soils, the pre-depositional ages of some of the compounds present in core GeoB23302-2 (up to 25 000 14C yr) are considerably greater than those previously attributed to permafrost-derived OM at other sites and at different timescales (e.g. up to ca. 10 000 14C yr; Gustafsson et al., 2011; Winterfeld et al., 2018). Although petrogenic contributions are commonly thought to be devoid of n-alkanoic acids, this assumption does not always hold (Kvenvolden, 1966), and the erosion of organic-rich sedimentary rocks can supply fossil OM to the ocean, thereby depleting the 14C compound-specific signal in the sediment (e.g. Raymond et al., 2004; Copard et al., 2007; Wu et al., 2022, and references therein). However, the OM present in core GeoB23302-2 presents δ13C values in the range from −25.9 ‰ to −22.3 ‰, heavier than the average value of δ13C = −29.4 ‰ displayed by organic-rich rocks in the region (e.g. Zhao et al., 2022). These values are rather comparable to those observed in peat, corroborating the results of the CPIalk and fββ proxies and pointing to a pre-aged immature source such as ancient peat. The pre-depositional ages observed during the peak of OM deposition likely result from a 14C reservoir effect, which is caused by radioactive decay and a lack of exchange with the atmosphere (Stuiver and Polach, 1977). This effect occurs as the OM is stored in its source reservoir before eventual erosion and transportation to the core location. Mechanisms such as deposition–resuspension loops on the continental slope could be invoked to explain pre-aged n-alkanoic acids during the most recent part of our record (Kusch et al., 2021, and references therein). To summarize, our results strongly support previous findings describing a massive remobilization of terrestrial C from the European continent to the North Atlantic Ocean during the last deglaciation, with notable peaks at ca. 19.5 and between approximately 19 and 17 cal kyr BP (Ménot et al., 2006). In addition, the set of proxies applied in the present study allows us to go further and suggest peat-derived material as a major source of OM to the Bay of Biscay during the last deglaciation.
4.2 Landscape development and OM remobilization mechanisms
Wetlands are dynamic ecosystems that fix CO2 from the atmosphere, store C and contribute to the C cycle through various processes, including the decomposition of OM that releases CO2 (Mitra et al., 2003). Therefore, the establishment of wetlands in the study region towards the end of the LGM and during the last deglaciation (Fig. 2d), combined with permafrost distribution data that imply gradual permafrost decomposition (e.g. Vandenberghe and Pissart, 1993; Levavasseur et al., 2011; Vandenberghe et al., 2012; Schaefer et al., 2014; Vandenberghe et al., 2014) and records of atmospheric CO2 concentration (Köhler et al., 2017), suggests the need to investigate thawing permafrost as a possible source of OM to the deposition site. Although some of the peatlands formed during the Eemian period were buried by glaciers and mineral sediments, Weichselian ice sheets were not as extensive as the ones from the Saalian, and some deposits remained uncovered (e.g. in northern Germany, on the cliff of the Elbe river; Ehlers et al., 2011). Peat deposits formed during the last interglacial occur widely across the studied region (Turner, 2000), from Belgium (e.g. De Moor, 1983) and the Netherlands (e.g. Schokker et al., 2004) to Poland (e.g. Woronko et al., 2018), through Germany (e.g. Börner et al., 2018; Grube and Usinger, 2017) and Denmark (e.g. Christiansen, 1998). Although the environmental conditions of the last glaciation were unfavourable for the development of peatlands, factors such as the formation of permafrost in Europe resulted in the long-term preservation of OM from older periods (e.g. frozen peat OM) (Treat et al., 2019). However, during the last deglaciation, the thawing of permafrost and the presence of meltwater streams may have contributed to the erosion of these peats. We propose that Eemian peatlands represent the primary source of fossil biomarkers transported to the Bay of Biscay, with processes such as thermal and physical erosion of these deposits (see e.g. Sidorchuk et al., 2009, 2011) leading to pre-aged material reaching the final burial site. At this point, it is crucial to emphasize that, in the context of this study, permafrost plays a more significant role as a storage mechanism for peatland OM rather than serving as a unique source of OM by itself.
In north-western and central Europe, continuous permafrost prevailed at approximately 27–17 cal kyr BP (Vandenberghe and Pissart, 1993), with the deposits likely degrading and shrinking due to the warming observed at the end of the LGM. For instance, in the Netherlands, there has been evidence of widespread permafrost degradation between 22 and 21 cal kyr BP (Van Huissteden et al., 2000), with continuous permafrost in the Dutch coversand region completely disappearing after 20 cal kyr BP (Bateman and Van Huissteden, 1999). This episode is an example of permafrost thawing that may have contributed to wetland development between ca. 21 and 16 cal kyr BP as recorded in our Paq record (Fig. 2d). Between 17 and 15 cal kyr BP, permafrost zones in the study region were restricted to areas near the retreating ice sheets (Renssen and Vandenberghe, 2003, and references therein). Afterwards, apart from a short later period of discontinuous permafrost (ca. 10.9–10.5 cal kyr BP) that has also been reported for north-western and central Europe (Vandenberghe and Pissart, 1993), there is evidence of the presence of permafrost in this region during the Younger Dryas (e.g. Isarin, 1997; Petera-Zganiacz and Dzieduszyńska, 2017).
The erosion of European permafrost and peatland deposits by glacial meltwater is a mechanism likely to have exported OM to the ocean. The decay of the European ice sheets and glaciers at the onset of the last deglaciation (e.g. Marks, 2002; Rinterknecht et al., 2006; Ó Cofaigh and Evans, 2007; Ballantyne and Ó Cofaigh, 2017; Patton et al., 2017) contributed to strengthening the Channel River discharge into the Bay of Biscay (Antoine et al., 2003; Bourillet et al., 2003), and it has been proposed that this was the main mechanism controlling the river activity during this time period (Toucanne et al., 2009). Deglacial pulses of meltwater emanating from the BIIS (at 22 and 18.6 cal kyr BP) and the FIS (starting around 19 cal kyr BP) (Bowen et al., 2002; Rinterknecht et al., 2006) were routed to the North Atlantic via the Channel River. In this way, the activity of the river responded to changes in the European ice masses, being particularly influenced by the dynamics of the FIS (Toucanne et al., 2010).
Although subglacial meltwater can flow through permeable sediments as groundwater, the presence of frozen ground with reduced hydraulic transmissivity, i.e. permafrost, hinders this process (Piotrowski, 1997). This leads to trapped pressurized water accumulating underneath ice sheets and eventually draining during catastrophic events. As the climate warmed, and the ice sheets retreated, and/or permafrost decayed, bursts of subglacial meltwater were released, carving glacial features known as tunnel valleys in the ground and discharging large amounts of eroded material into rivers (Piotrowski, 1994, 1999; Kirkham et al., 2022; and references therein). Subglacial channels from major Pleistocene glaciations are still present today in Europe and serve as evidence of this phenomenon (e.g. Piotrowski, 1999; Piotrowski et al., 1999). Meltwater streams from the FIS discharged through the Elbe river and provoked several flooding events of the Channel River (Mangerud et al., 2004; Toucanne et al., 2015), with remarkable episodes (R2–R5) recorded as peaks in the ratios Ti Ca and Fe Ca of both the GeoB23302-2 and the MD95 2002 archives (Fig. S3). Floods R2–R5 were most likely associated with enhanced processes of erosion and sediment export in the catchment (see e.g. Bogen and Bønsnes, 2003), and the intensified freshwater influx, resulting from riverine discharge due to a mixture of precipitation and glacial meltwaters, is reflected in the terrestrially sourced OM signal shown by our BIT index record, which corroborates that reported for core MD95 2002 (Ménot et al., 2006) (Fig. S4). Furthermore, the process of post-glacial sea-level rise may have played a role in the erosion of coastal permafrost deposits, potentially serving as an additional pathway for the transport of OM to the ocean (e.g. Meyer et al., 2019).
The remobilization of OM from land to ocean is largely mediated by rivers, with factors such as precipitation and temperature being major regulators of fluvial C fluxes (Bauer et al., 2013, and references therein). Between 21 and 17 cal kyr BP, a temperature rise (Fig. 2a) observed in various Atlantic environmental records (e.g. Arz et al., 1999; Bard et al., 2000; Combourieu Nebout et al., 2002; Pailler and Bard, 2002) marked a transition from cold and dry to warm and wet conditions in continental Europe. For example, a gradual increase in the north-eastern Atlantic sea surface temperature (SST) starting at about 25 cal kyr BP and preceding the peak of terrigenous deposition is recorded in core SU8118 (37∘46′ N, 10∘11′ W) (Bard et al., 2000). This same warming trend can be observed on land, reflected in the δ13C signature of a speleothem record from western Europe (45∘30′ N, 0∘50′ E) (Wainer et al., 2011) and starting roughly at the same time (Fig. 2). The speleothem time series is not high-resolution, and long-term trends may be in fact punctuated with short-term oscillations. In any case, it is important to acknowledge that, although speleothem δ13C values can potentially serve as a proxy for temperature (see e.g. Lechleitner et al., 2021, and references therein), the correlation must be interpreted with caution due to several other factors influencing C isotopic ratios in these archives (Fohlmeister et al., 2020). Enhanced precipitation in response to warming led to increases in fluvial runoff and, due to widespread permafrost, increased erosion and transport of sediment from land to the Channel River outlet (Ménot et al., 2006). After approximately 18 cal kyr BP, as the climate warmed, the area occupied by peatlands in Europe increased (Müller and Joos, 2020). This is in agreement with our Paq index record, which indicates the re-establishment of formerly frozen wetlands, potentially including peat-rich environments (Fig. 2d).
Considering that the OM buried in marine sediment is only a relatively small part of the total OM entering rivers, which is predominantly returned to the atmosphere as CO2 (e.g. Aufdenkampe et al., 2011), the OM export to the Bay of Biscay via the Channel River is likely to have been accompanied by the transfer of CO2 and CH4 to the atmosphere (e.g. Schneider Von Deimling et al., 2015; Schuur et al., 2015; Bröder et al., 2018). It follows that our comprehensive analysis, encompassing biomarkers, elemental proxies and radiocarbon dating, consistently corroborates the hypothesis of permafrost thawing in the Northern Hemisphere contributing to the observed perturbations in the atmospheric C reservoir (Köhler et al., 2014). This essentially means that north-western and central Europe too, similar to other permafrost sites (Winterfeld et al., 2018; Meyer et al., 2019), may have contributed to the deglacial rise in atmospheric CO2 (Köhler et al., 2014; Marcott et al., 2014). However, it is likely that the deglacial loss of European permafrost was offset by the subsequent accumulation of significant amounts of C in permafrost-free soils and peatlands (Lindgren et al., 2018). Our elevated pre-depositional ages at ca. 17.5 cal kyr BP (up to ca. 15 14C kyr) may partly explain the steep drop in the 14C signature of atmospheric CO2 during the period known as the Mystery Interval (17.5–14.5 kyr BP) (see e.g. Broecker and Barker, 2007). In other words, this result implies that thawing European permafrost combined with the deep-ocean reservoir contributed 14C-depleted CO2 to the atmosphere during this period. However, our results show that the remobilization of C from this terrestrial pool started as early as ca. 20.2 cal kyr BP, which considerably precedes estimates of large permafrost contributions to atmospheric CO2 between 17.5 and 15 kyr BP (Crichton et al., 2016). This suggests the need to investigate leads and lags in the permafrost carbon feedback.
After approximately 18 cal kyr BP, a rerouting of the Elbe–Weser system meant that FIS meltwater carrying ancient C was being delivered to the Norwegian Channel (Toucanne et al., 2010, and references therein). After 17 cal kyr BP, sea-level rise caused a shift in the shoreline, with the Bay of Biscay no longer being suitable to record terrestrial runoff during the Holocene (Lambeck, 1997). This is reflected in the sudden drop observed in the BIT index record (Fig. 2f). Notably, although our data support what has been previously inferred for the study region (Ménot et al., 2006; Rostek and Bard, 2013; Soulet et al., 2013), the distinct timing for the discharge peak observed in this study compared to other sites may imply different mechanisms of C remobilization, and these need to be further investigated. Indeed, factors such as the local hydrology and vegetation have been shown to play a role in the accumulation and degradation pathways of permafrost-influenced peatlands (Hugelius et al., 2020, and references therein).
Peat-forming wetlands remain an important source of terrestrial OM to the ocean and of CH4 and CO2 to the atmosphere, with flux rates likely increasing due to current warming (Freeman et al., 2001; Hodgkins et al., 2014). In high-northern-latitude wetlands, it has been shown that permafrost degradation leads to wetland shrinkage (Avis et al., 2011). In the tropics the situation is also critical, with anthropogenic (Moore et al., 2013) and natural (Schefuß et al., 2016; Garcin et al., 2022) factors contributing to the remobilization of pre-aged C from peatlands. Therefore, the release of large amounts of peat-derived OM described here for deglacial Europe has analogues in the present day and may be useful to inform future projections of permafrost peatland loss (e.g. Fewster et al., 2022), with our results advocating for the importance of better constraining the C cycle in wetlands.
To reconcile the great pre-depositional ages observed here with geochemical data that do not hint towards highly degraded petrogenic material, we argue that the OM in core GeoB23302-2 is mostly derived from ancient continental peat deposits. During the last interglacial, peatlands were established in the European landscape. These deposits were widely distributed and were preserved in a frozen state throughout the last glaciation due to the widespread presence of permafrost. Over the course of the last deglaciation, warming and episodes of ice-sheet retreat and associated flooding through the Channel River resulted in the erosion of these permafrost deposits, enhancing the downstream transport of sediment and mobilizing ancient C to the core site. Our results indicate that during the period between 20.2 and 15.8 cal kyr BP, a substantial portion of the OM transported to the Bay of Biscay originated from ancient European peatlands. After approximately 17 cal kyr BP, our core location was not suitable for recording terrigenous inputs via the Channel River. Instead, the Norwegian Channel may have become the primary recipient of fluvially discharged permafrost-derived C. It is possible that the emission of greenhouse gases resulting from the degradation of formerly frozen OM in European permafrost contributed to the rapid rise of approximately 30 ppm in the atmospheric CO2 concentration between 17.5 and 16 cal kyr BP. However, further investigation is needed to accurately quantify the rates and magnitudes of the processes responsible for this contribution. This study provides empirical evidence of a cycle of peat formation during warm periods and long-term storage under colder conditions. Owing to the size of the C pool involved, such a mechanism is likely to increase atmospheric greenhouse gas concentrations, with important implications for Earth’s climate. In this context, our results will be useful to better constrain the role of ancient C mobilization and the permafrost carbon feedback in climate models.
Data generated in this study are freely available at https://doi.pangaea.de/10.1594/PANGAEA.954937 (Queiroz Alves et al., 2023).
The supplement related to this article is available online at: https://doi.org/10.5194/cp-20-121-2024-supplement.
GM designed the study. GM, KZ and HG collected the sediment core. Funding for the project was acquired by GM and EQA. EQA and WW conducted the laboratory analyses with the support of JH, HG, TG and TT. EQA, GM, JH, HG and WW analysed the data. EQA wrote the paper and generated the figures. All authors provided feedback on the paper.
One of the co-authors is an employee of EGU. This has not influenced the decision of the independent journal editor.
Publisher’s note: Copernicus Publications remains neutral with regard to jurisdictional claims made in the text, published maps, institutional affiliations, or any other geographical representation in this paper. While Copernicus Publications makes every effort to include appropriate place names, the final responsibility lies with the authors.
This research was funded by the Alexander von Humboldt Foundation via a post-doctoral fellowship granted to Eduardo Queiroz Alves. Thanks are also due to the Brazilian National Council for Scientific and Technological Development (CNPq) for the support provided to Eduardo Queiroz Alves. Hendrik Grotheer was funded by the German Science Foundation within the Cluster of Excellence EXC 2077 “The Oceans Floor – Earth’s Uncharted Interface” (project no. 390741603). We thank Enno Schefuß, Kita Macario and Fernanda Matos for helpful discussions that benefited this research. We are also grateful for the data provided by Samuel Toucanne and Fortunat Joos and for the technical laboratory support offered by Elizabeth Bonk and Lea Phillips. Finally, we are grateful to Alberto Reyes, Benjamin Gagliotti, and two anonymous referees for their constructive comments and valuable suggestions.
This research has been supported by the Alexander von Humboldt-Stiftung (grant no. 1208249) and the German Science Foundation within the Cluster of Excellence EXC 2077 “The Oceans Floor – Earth’s Uncharted Interface” (project no. 390741603).
This paper was edited by Alberto Reyes and reviewed by Benjamin Gaglioti and two anonymous referees.
Andersen, K. K., Azuma, N., Barnola, J. M., Bigler, M., Biscaye, P., Caillon, N., Chappellaz, J., Clausen, H. B., Dahl-Jensen, D., Fischer, H., Flückiger, J., Fritzsche, D., Fujii, Y., Goto-Azuma, K., Grønvold, K., Gundestrup, N. S., Hansson, M., Huber, C., Hvidberg, C. S., Johnsen, S. J., Jonsell, U., Jouzel, J., Kipfstuhl, S., Landais, A., Leuenberger, M., Lorrain, R., Masson-Delmotte, V., Miller, H., Motoyama, H., Narita, H., Popp, T., Rasmussen, S. O., Raynaud, D., Rothlisberger, R., Ruth, U., Samyn, D., Schwander, J., Shoji, H., Siggard-Andersen, M. L., Steffensen, J. P., Stocker, T., Sveinbjörnsdóttir, A. E., Svensson, A., Takata, M., Tison, J. L., Thorsteinsson, T., Watanabe, O., Wilhelms, F., and White, J. W.: High-resolution record of Northern Hemisphere climate extending into the last interglacial period, Nature, 431, 147–151, 2004. a
Antoine, P., Coutard, J. P., Gibbard, P., Hallegouet, B., Lautridou, J. P., and Ozouf, J. C.: The Pleistocene rivers of the English Channel region, J. Quaternary Sci., 18, 227–243, 2003. a, b
Arz, H. W., Pätzold, J., and Wefer, G.: Climatic changes during the last deglaciation recorded in sediment cores from the northeastern Brazilian Continental Margin, Geo-Mar. Lett., 19, 209–218, 1999. a, b
Aufdenkampe, A. K., Mayorga, E., Raymond, P. A., Melack, J. M., Doney, S. C., Alin, S. R., Aalto, R. E., and Yoo, K.: Riverine coupling of biogeochemical cycles between land, oceans, and atmosphere, Front. Ecol. Environ., 9, 53–60, 2011. a
Avis, C. A., Weaver, A. J., and Meissner, K. J.: Reduction in areal extent of high-latitude wetlands in response to permafrost thaw, Nat. Geosci., 4, 444–448, 2011. a
Ballantyne, C. K. and Ó Cofaigh, C.: The Last Irish Ice Sheet: Extent and Chronology, in: Advances in Irish Quaternary Studies, Atlantis Press, Paris, 101–149, https://doi.org/10.3318/ijes.2018.36.4, 2017. a
Bard, E., Rostek, F., Turon, J. L., and Gendreau, S.: Hydrological impact of Heinrich events in the subtropical Northeast Atlantic, Science, 289, 1321–1324, 2000. a, b, c
Bateman, M. D. and Van Huissteden, J.: The timing of last-glacial periglacial and aeolian events, Twente, eastern Netherlands, J. Quaternary Sci., 14, 277–283, 1999. a
Bauer, J. E., Cai, W. J., Raymond, P. A., Bianchi, T. S., Hopkinson, C. S., and Regnier, P. A.: The changing carbon cycle of the coastal ocean, Nature, 504, 61–70, 2013. a
Bauska, T. K., Baggenstos, D., Brook, E. J., Mix, A. C., Marcott, S. A., Petrenko, V. V., Schaefer, H., Severinghaus, J. P., Lee, J. E., and Thiemens, M. H.: Carbon isotopes characterize rapid changes in atmospheric carbon dioxide during the last deglaciation, P. Natl. Acad. Sci. USA, 113, 3465–3470, 2016. a
Bianchi, T. and Canuel, E.: Chemical biomarkers in aquatic ecosystems, Princeton University Press, ISBN 9780691134147, 2011. a, b
Bogen, J. and Bønsnes, T. E.: Erosion and sediment transport in High Arctic rivers, Svalbard, Polar Res., 22, 175–189, 2003. a
Börner, A., Hrynowiecka, A., Stachowicz-Rybka, R., Niska, M., Moskal-del Hoyo, M., Kuznetsov, V., Maksimov, F., and Petrov, A.: Palaeoecological investigations and 230Th U dating of the Eemian Interglacial peat sequence from Neubrandenburg-Hinterste Mühle (Mecklenburg-Western Pomerania, NE Germany), Quatern. Int., 467, 62–78, 2018. a
Bourillet, J. F., Reynaud, J. Y., Baltzer, A., and Zaragosi, S.: The “Fleuve Manche”: The submarine sedimentary features from the outer shelf to the deep-sea fans, J. Quaternary Sci., 18, 261–282, 2003. a, b
Bowen, D. Q., Phillips, F. M., McCabe, A. M., Knutz, P. C., and Sykes, G. A.: New data for the Last Glacial Maximum in Great Britain and Ireland, Quaternary Sci. Rev., 21, 89–101, 2002. a, b
Bray, E. E. and Evans, E. D.: Distribution of n-paraffins as a clue to recognition of source beds, Geochim. Cosmochim. Ac., 22, 2–15, 1961. a, b, c, d
Bröder, L., Tesi, T., Andersson, A., Semiletov, I., and Gustafsson, Ö.: Bounding cross-shelf transport time and degradation in Siberian-Arctic land-ocean carbon transfer, Nat. Commun., 9, 806, https://doi.org/10.1038/s41467-018-03192-1, 2018. a, b
Broecker, W. and Barker, S.: A 190 ‰ drop in atmosphere's Δ14C during the “Mystery Interval” (17.5 to 14.5 kyr), Earth Planet. Sc. Lett., 256, 90–99, 2007. a
Bronk Ramsey, C.: Radiocarbon calibration and analysis of stratigraphy: the OxCal program, Radiocarbon, 37, 425–430, 1995. a, b
Bronk Ramsey, C.: Deposition models for chronological records, Quaternary Sci. Rev., 27, 42–60, 2008. a
Bronk Ramsey, C.: Bayesian analysis of radiocarbon dates, Radiocarbon, 51, 337–360, 2009a. a, b
Bronk Ramsey, C.: Dealing with outliers and offsets in radiocarbon dating, Radiocarbon, 51, 1023–1045, 2009b. a
Bronk Ramsey, C. and Lee, S.: Recent and Planned Developments of the Program OxCal, Radiocarbon, 55, 720–730, 2013. a
Christiansen, H. H.: Periglacial sediments in an Eemian-Weichselian succession at Emmerlev Klev, southwestern Jutland, Denmark, Palaeogeogr. Palaeocl., 138, 245–258, 1998. a
Ciais, P., Tagliabue, A., Cuntz, M., Bopp, L., Scholze, M., Hoffmann, G., Lourantou, A., Harrison, S. P., Prentice, I. C., Kelley, D. I., Koven, C., and Piao, S. L.: Large inert carbon pool in the terrestrial biosphere during the Last Glacial Maximum, Nat. Geosci., 5, 74–79, 2012. a
Combourieu Nebout, N., Turon, J. L., Zahn, R., Capotondi, L., Londeix, L., and Pahnke, K.: Enhanced aridity and atmospheric high-pressure stability over the western Mediterranean during the North Atlantic cold events of the past 50 k.y, Geology, 30, 863–866, 2002. a
Copard, Y., Amiotte-Suchet, P., and Di-Giovanni, C.: Storage and release of fossil organic carbon related to weathering of sedimentary rocks, Earth Planet. Sc. Lett., 258, 345–357, 2007. a
Crichton, K. A., Bouttes, N., Roche, D. M., Chappellaz, J., and Krinner, G.: Permafrost carbon as a missing link to explain CO 2 changes during the last deglaciation, Nat. Geosci., 9, 683–686, 2016. a, b
Crivellari, S., Chiessi, C. M., Kuhnert, H., Häggi, C., da Costa Portilho-Ramos, R., Zeng, J. Y., Zhang, Y., Schefuß, E., Mollenhauer, G., Hefter, J., Alexandre, F., Sampaio, G., and Mulitza, S.: Increased Amazon freshwater discharge during late Heinrich Stadial 1, Quaternary Sci. Rev., 181, 144–155, 2018. a
De Moor, G.: Cryogenic Structures in the Weichselian Deposits of Northern Belgium and their Significance, Polarforschung, 53, 79–86, 1983. a
Dickson, A. J., Leng, M. J., Maslin, M. A., and Röhl, U.: Oceanic, atmospheric and ice-sheet forcing of South East Atlantic Ocean productivity and South African monsoon intensity during MIS-12 to 10, Quaternary Sci. Rev., 29, 3936–3947, https://doi.org/10.1016/j.quascirev.2010.09.014, 2010. a
Dypvik, H. and Harris, N. B.: Geochemical facies analysis of fine-grained siliciclastics using Th U, Zr Rb and (Zr + Rb) Sr ratios, Chem. Geol., 181, 131–146, 2001. a
Eglinton, T. I., Aluwihare, L. I., Bauer, J. E., Druffel, E. R., and McNichol, A. P.: Gas chromatographic isolation of individual compounds from complex matrices for radiocarbon dating, Anal. Chem., 68, 904–912, 1996. a
Ehlers, J., Grube, A., Stephan, H. J., and Wansa, S.: Pleistocene glaciations of North Germany-New results, Developments in Quaternary Science, 15, 149–162, 2011. a
Fairbanks, R. G.: A 17,000-year glacio-eustatic sea level record: influence of glacial melting rates on the Younger Dryas event and deep-ocean circulation, Nature, 342, 637–642, 1989. a
Farrington, J. W. and Tripp, B. W.: Hydrocarbons in western North Atlantic surface sediments, Geochim. Cosmochim. Ac., 41, 1627–1641, 1977. a
Feurdean, A., Grindean, R., Florescu, G., Tanţău, I., Niedermeyer, E. M., Diaconu, A.-C., Hutchinson, S. M., Nielsen, A. B., Sava, T., Panait, A., Braun, M., and Hickler, T.: The transformation of the forest steppe in the lower Danube Plain of southeastern Europe: 6000 years of vegetation and land use dynamics, Biogeosciences, 18, 1081–1103, https://doi.org/10.5194/bg-18-1081-2021, 2021. a
Fewster, R. E., Morris, P. J., Ivanovic, R. F., Swindles, G. T., Peregon, A. M., and Smith, C. J.: Imminent loss of climate space for permafrost peatlands in Europe and Western Siberia, Nat. Clim. Change, 12, 373–379, 2022. a
Ficken, K. J., Li, B., Swain, D. L., and Eglinton, G.: An n-alkane proxy for the sedimentary input of submerged/floating freshwater aquatic macrophytes, Org. Geochem., 31, 745–749, 2000. a, b, c
Fohlmeister, J., Voarintsoa, N. R. G., Lechleitner, F. A., Boyd, M., Brandtstätter, S., Jacobson, M. J., and Oster, J. L.: Main controls on the stable carbon isotope composition of speleothems, Geochim. Cosmochim. Ac., 279, 67–87, 2020. a
Freeman, C., Evans, C., and Monteih, D.: Export of organic carbon from peat soils, Nature, 412, 785, https://doi.org/10.1038/35090628, 2001. a
Garcin, Y., Schefuß, E., Dargie, G. C., Hawthorne, D., Ifo, S. A., Wenina, Y. E. M., and Mbemba, M.: Hydroclimatic vulnerability of peat carbon in the central Congo Basin, Nature, 612, 277–282, https://doi.org/10.1038/s41586-022-05389-3, 2022. a, b
Gibbard, P. L.: The history of the great northwest European rivers during the past three million years, Philos. T. R. Soc. B, 318, 559–602, 1988. a
Grotheer, H., Meyer, V., Riedel, T., Pfalz, G., Mathieu, L., Hefter, J., Gentz, T., Lantuit, H., Mollenhauer, G., and Fritz, M.: Burial and Origin of Permafrost-Derived Carbon in the Nearshore Zone of the Southern Canadian Beaufort Sea, Geophys. Res. Lett., 47, e2019GL085897, https://doi.org/10.1029/2019GL085897, 2020. a
Grube, A. and Usinger, H.: Spring fed raised peat hummocks with tufa deposits at the Farbeberg hills (Northwest-Germany): Structure, genesis and paleoclimatic conclusions (Eemian, Holocene), E and G Quaternary Science Journal, 66, 14–31, 2017. a
Gustafsson, Ö., van Dongen, B. E., Vonk, J. E., Dudarev, O. V., and Semiletov, I. P.: Widespread release of old carbon across the Siberian Arctic echoed by its large rivers, Biogeosciences, 8, 1737–1743, https://doi.org/10.5194/bg-8-1737-2011, 2011. a
He, D., Nemiah Ladd, S., Saunders, C. J., Mead, R. N., and Jaffé, R.: Distribution of n-alkanes and their δ2H and δ13C values in typical plants along a terrestrial-coastal-oceanic gradient, Geochim. Cosmochim. Ac., 281, 31–52, 2020. a
Heaton, T. J., Köhler, P., Butzin, M., Bard, E., Reimer, R. W., Austin, W. E., Bronk Ramsey, C., Grootes, P. M., Hughen, K. A., Kromer, B., Reimer, P. J., Adkins, J., Burke, A., Cook, M. S., Olsen, J., and Skinner, L. C.: Marine20 – The Marine Radiocarbon Age Calibration Curve (0–55,000 cal BP), Radiocarbon, 62, 779–820, 2020. a, b
Heaton, T. J., Bard, E., Bronk Ramsey, C., Butzin, M., Hatté, C., Hughen, K. A., Köhler, P., and Reimer, P. J.: a Response To Community Questions on the Marine20 Radiocarbon Age Calibration Curve: Marine Reservoir Ages and the Calibration of 14C Samples From the Oceans, Radiocarbon, 65, 247–273, 2023. a
Hefter, J.: Analysis of Alkenone Unsaturation Indices with Fast Gas Chromatography/Time-of-Flight Mass Spectrometry, Anal. Chem., 80, 2161–2170, 2008. a
Herfort, L., Schouten, S., Boon, J. P., Woltering, M., Baas, M., Weijers, J. W., and Sinninghe Damsté, J. S.: Characterization of transport and deposition of terrestrial organic matter in the southern North Sea using the BIT index, Limnol. Oceanogr., 51, 2196–2205, 2006. a
Hodgkins, S. B., Tfaily, M. M., McCalley, C. K., Logan, T. A., Crill, P. M., Saleska, S. R., Rich, V. I., and Chanton, J. P.: Changes in peat chemistry associated with permafrost thaw increase greenhouse gas production, P. Natl. Acad. Sci. USA, 111, 5819–5824, https://doi.org/10.1073/pnas.1314641111, 2014. a
Hopmans, E. C., Weijers, J. W., Schefuß, E., Herfort, L., Sinninghe Damsté, J. S., and Schouten, S.: A novel proxy for terrestrial organic matter in sediments based on branched and isoprenoid tetraether lipids, Earth Planet. Sc. Lett., 224, 107–116, 2004. a, b, c
Hopmans, E. C., Schouten, S., and Sinninghe Damsté, J. S.: The effect of improved chromatography on GDGT-based palaeoproxies, Org. Geochem., 93, 1–6, 2016. a
Hugelius, G., Strauss, J., Zubrzycki, S., Harden, J. W., Schuur, E. A. G., Ping, C.-L., Schirrmeister, L., Grosse, G., Michaelson, G. J., Koven, C. D., O'Donnell, J. A., Elberling, B., Mishra, U., Camill, P., Yu, Z., Palmtag, J., and Kuhry, P.: Estimated stocks of circumpolar permafrost carbon with quantified uncertainty ranges and identified data gaps, Biogeosciences, 11, 6573–6593, https://doi.org/10.5194/bg-11-6573-2014, 2014. a, b
Hugelius, G., Loisel, J., Chadburn, S., Jackson, R. B., Jones, M., MacDonald, G., Marushchak, M., Olefeldt, D., Packalen, M., Siewert, M. B., Treat, C., Turetsky, M., Voigt, C., and Yu, Z.: Large stocks of peatland carbon and nitrogen are vulnerable to permafrost thaw, P. Natl. Acad. Sci. USA, 117, 20438–20446, 2020. a
Inglis, G. N., Naafs, B. D. A., Zheng, Y., McClymont, E. L., Evershed, R. P., and Pancost, R. D.: Distributions of geohopanoids in peat: Implications for the use of hopanoid-based proxies in natural archives, Geochim. Cosmochim. Ac., 224, 249–261, https://doi.org/2017.12.029, 2018. a
Isarin, R. F.: Permafrost distribution and temperatures in Europe during the Younger Dryas, Permafrost Periglac., 8, 313–333, 1997. a
Itambi, A. C., Von Dobeneck, T., Mulitza, S., Bickert, T., and Heslop, D.: Millennial-scale northwest African droughts related to Heinrich events and Dansgaard-Oeschger cycles: Evidence in marine sediments from offshore Senegal, Paleoceanography, 24, 1–16, 2009. a
Jeng, W. L.: Higher plant n-alkane average chain length as an indicator of petrogenic hydrocarbon contamination in marine sediments, Mar. Chem., 102, 242–251, 2006. a
Jennerjahn, T. C., Ittekkot, V., Arz, H. W., Behling, H., Pätzold, J., and Wefer, G.: Asynchronous terrestrial and marine signals of climate change during Heinrich events, Science, 306, 2236–2239, 2004. a
Keskitalo, K., Tesi, T., Bröder, L., Andersson, A., Pearce, C., Sköld, M., Semiletov, I. P., Dudarev, O. V., and Gustafsson, Ö.: Sources and characteristics of terrestrial carbon in Holocene-scale sediments of the East Siberian Sea, Clim. Past, 13, 1213–1226, https://doi.org/10.5194/cp-13-1213-2017, 2017. a
Kim, J. H., Schouten, S., Bonnin, J., Buscail, R., Ludwig, W., Sinninghe Damsté, J. S., and Bourrin, F.: Origin and distribution of terrestrial organic matter in the NW Mediterranean (Gulf of Lions): Exploring the newly developed BIT index, Geochem. Geophy. Geosy., 7, Q11017, https://doi.org/10.1029/2006GC001306, 2006. a
Kirkham, J. D., Hogan, K. A., Larter, R. D., Arnold, N. S., Ely, J. C., Clark, C. D., Self, E., Games, K., Huuse, M., Stewart, M. A., Ottesen, D., and Dowdeswell, J. A.: Tunnel valley formation beneath deglaciating mid-latitude ice sheets : Observations and modelling, Quaternary Sci. Rev., 323, 107680, https://doi.org/10.1016/j.quascirev.2022.107680, 2022. a
Köhler, P., Knorr, G., and Bard, E.: Permafrost thawing as a possible source of abrupt carbon release at the onset of the Bølling/Allerød, Nat. Commun., 5, 5520, https://doi.org/10.1038/ncomms6520, 2014. a, b, c, d
Köhler, P., Nehrbass-Ahles, C., Schmitt, J., Stocker, T. F., and Fischer, H.: A 156 kyr smoothed history of the atmospheric greenhouse gases CO2, CH4, and N2O and their radiative forcing, Earth Syst. Sci. Data, 9, 363–387, https://doi.org/10.5194/essd-9-363-2017, 2017. a, b
Koppes, M. and Hallet, B.: Erosion rates during rapid deglaciation in Icy Bay, Alaska, J. Geophys. Res.-Earth, 111, F02023, https://doi.org/10.1029/2005JF000349, 2006. a
Koppes, M. N. and Hallet, B.: Influence of rapid glacial retreat on the rate of erosion by tidewater glaciers, Geology, 30, 47–50, 2002. a
Kusch, S., Rethemeyer, J., Schefuß, E., and Mollenhauer, G.: Controls on the age of vascular plant biomarkers in Black Sea sediments, Geochim. Cosmochim. Ac., 74, 7031–7047, 2010. a
Kusch, S., Mollenhauer, G., Willmes, C., Hefter, J., Eglinton, T. I., and Galy, V.: Controls on the age of plant waxes in marine sediments – A global synthesis, Org. Geochem., 157, 104259, https://doi.org/10.1016/j.orggeochem.2021.104259, 2021. a
Kvenvolden, K. A.: Molecular Distributions of Normal Fatty Acids and Paraffins in Some Lower Cretaceous Sediments, Nature, 209, 573–577, https://doi.org/10.1038/209573a0, 1966. a
Kylander, M. E., Ampel, L., Wohlfarth, B., and Veres, D.: High-resolution X-ray fluorescence core scanning analysis of Les Echets (France) sedimentary sequence: New insights from chemical proxies, J. Quaternary Sci., 26, 109–117, 2011. a
Tisnérat-Laborde, N., Paterne, M., Métivier, B., Arnold, M., Yiou, P., Blamart, D., and Raynaud, S.: Variability of the northeast Atlantic sea surface Δ14C and marine reservoir age and the North Atlantic Oscillation (NAO), Quaternary Sci. Rev., 29, 2633–2646, https://doi.org/10.1016/j.quascirev.2010.06.013, 2010. a
Lambeck, K.: Sea-level change along the French Atlantic and Channel coasts since the time of the Last Glacial Maximum, Palaeogeogr. Palaeocl., 129, 1–22, 1997. a, b
Lambeck, K., Rouby, H., Purcell, A., Sun, Y., and Sambridge, M.: Sea level and global ice volumes from the Last Glacial Maximum to the Holocene, P. Natl. Acad. Sci. USA, 111, 15296–15303, 2014. a
Lebreiro, S. M., Voelker, A. H., Vizcaino, A., Abrantes, F. G., Alt-Epping, U., Jung, S., Thouveny, N., and Gràcia, E.: Sediment instability on the Portuguese continental margin under abrupt glacial climate changes (last 60 kyr), Quaternary Sci. Rev., 28, 3211–3223, 2009. a
chleitner, F. A., Day, C. C., Kost, O., Wilhelm, M., Haghipour, N., Henderson, G. M., and Stoll, H. M.: Stalagmite carbon isotopes suggest deglacial increase in soil respiration in western Europe driven by temperature change, Clim. Past, 17, 1903–1918, https://doi.org/10.5194/cp-17-1903-2021, 2021. a
Levavasseur, G., Vrac, M., Roche, D. M., Paillard, D., Martin, A., and Vandenberghe, J.: Present and LGM permafrost from climate simulations: contribution of statistical downscaling, Clim. Past, 7, 1225–1246, https://doi.org/10.5194/cp-7-1225-2011, 2011. a, b
Lindgren, A., Hugelius, G., and Kuhry, P.: Extensive loss of past permafrost carbon but a net accumulation into present-day soils, Nature, 560, 219–222, 2018. a
Liu, X.-l., Lipp, J. S., Simpson, J. H., Lin, Y.-s., Summons, R. E., and Hinrichs, K.-U.: Mono- and dihydroxyl glycerol dibiphytanyl glycerol tetraethers in marine sediments : Identification of both core and intact polar lipid forms, Geochim. Cosmochim. Ac., 89, 102–115, 2012. a
Mangerud, J., Jakobsson, M., Alexanderson, H., Astakhov, V., Clarke, G. K., Henriksen, M., Hjort, C., Krinner, G., Lunkka, J. P., Möller, P., Murray, A., Nikolskaya, O., Saarnisto, M., and Svendsen, J. I.: Ice-dammed lakes and rerouting of the drainage of northern Eurasia during the Last Glaciation, Quaternary Sci. Rev., 23, 1313–1332, 2004. a, b
Marcott, S. A., Bauska, T. K., Buizert, C., Steig, E. J., Rosen, J. L., Cuffey, K. M., Fudge, T. J., Severinghaus, J. P., Ahn, J., Kalk, M. L., McConnell, J. R., Sowers, T., Taylor, K. C., White, J. W., and Brook, E. J.: Centennial-scale changes in the global carbon cycle during the last deglaciation, Nature, 514, 616–619, 2014. a
Marks, L.: Last Glacial Maximum in Poland, Quaternary Sci. Rev., 21, 103–110, 2002. a
Martens, J., Wild, B., Pearce, C., Tesi, T., Andersson, A., Bröder, L., O'Regan, M., Jakobsson, M., Sköld, M., Gemery, L., Cronin, T. M., Semiletov, I., Dudarev, O. V., and Gustafsson, Ö.: Remobilization of Old Permafrost Carbon to Chukchi Sea Sediments During the End of the Last Deglaciation, Global Biogeochem. Cy., 33, 2–14, 2019. a
Martens, J., Wild, B., Muschitiello, F., O'Regan, M., Jakobsson, M., Semiletov, I., Dudarev, O. V., and Gustafsson, Ö.: Remobilization of dormant carbon from Siberian-Arctic permafrost during three past warming events, Science Advances, 6, eabb6546, https://doi.org/10.1126/sciadv.abb6546, 2020. a
Marzi, R., Torkelson, B. E., and Olson, R. K.: A revised carbon preference index, Org. Geochem., 20, 1303–1306, 1993. a, b, c
Ménot, G., Bard, E., Rostek, F., Weijers, J. W. H., Hopmans, E. C., Schouten, S., and Damste, J. S. S.: Early Reactivation of European Rivers During the Last Deglaciation, Science, 313, 1623–1625, 2006. a, b, c, d, e, f, g, h, i, j
Meyers and Ishiwatari, R.: Lacustrine organic geochemistry-an overview of indicators of organic matter sources and diagenesis in lake sediments, Org. Geochem., 20, 867–900, 1993. a
Meyer, V. D., Hefter, J., Köhler, P., Tiedemann, R., Gersonde, R., Wacker, L., and Mollenhauer, G.: Permafrost-carbon mobilization in Beringia caused by deglacial meltwater runoff, sea-level rise and warming, Environ. Res. Lett., 14, 085003, https://doi.org/10.1088/1748-9326/ab2653, 2019. a, b, c, d, e, f, g
Mitra, S., Wassmann, R., and Vlek, P. L. G.: Global inventory of wetlands and their role in the carbon cycle, ZEF Discusson Paper on Development Policy, Bonn, p. 57, https://doi.org/10.22004/ag.econ.18771, 2003. a
Mollenhauer, G., Grotheer, H., Gentz, T., Bonk, E., and Hefter, J.: Standard operation procedures and performance of the MICADAS radiocarbon laboratory at Alfred Wegener Institute (AWI), Germany, Nucl. Instrum. Meth. B, 496, 45–51, https://doi.org/10.1016/j.nimb.2021.03.016, 2021. a
Moore, S., Evans, C. D., Page, S. E., Garnett, M. H., Jones, T. G., Freeman, C., Hooijer, A., Wiltshire, A. J., Limin, S. H., and Gauci, V.: Deep instability of deforested tropical peatlands revealed by fluvial organic carbon fluxes, Nature, 493, 660–663, https://doi.org/10.1038/nature11818, 2013. a
Müller, J. and Joos, F.: Global peatland area and carbon dynamics from the Last Glacial Maximum to the present – a process-based model investigation, Biogeosciences, 17, 5285–5308, https://doi.org/10.5194/bg-17-5285-2020, 2020. a, b
Nichols, J. E., Booth, R. K., Jackson, S. T., Pendall, E. G., and Huang, Y.: Paleohydrologic reconstruction based on n-alkane distributions in ombrotrophic peat, Org. Geochem., 37, 1505–1513, 2006. a
Nieuwenhuize, J., Maas, Y., and Middelburg, J.: Rapid analysis of organic carbon and nitrogen in particulate materials, Mar. Chem., 45, 217–224, 1994. a
Ó Cofaigh, C. and Evans, D. J.: Radiocarbon constraints on the age of the maximum advance of the British-Irish Ice Sheet in the Celtic Sea, Quaternary Sci. Rev., 26, 1197–1203, 2007. a
Pailler, D. and Bard, E.: High frequency palaeoceanographic changes during the past 140 000 yr recorded by the organic matter in sediments of the Iberian Margin, Palaeogeogr. Palaeocl., 181, 431–452, 2002. a
Patton, H., Hubbard, A., Andreassen, K., Auriac, A., Whitehouse, P. L., Stroeven, A. P., Shackleton, C., Winsborrow, M., Heyman, J., and Hall, A. M.: Deglaciation of the Eurasian ice sheet complex, Quaternary Sci. Rev., 169, 148–172, 2017. a, b
Perez, L., García-Rodríguez, F., and Hanebuth, T. J. J.: Variability in terrigenous sediment supply offshore of the Río de la Plata (Uruguay) recording the continental climatic history over the past 1200 years, Clim. Past, 12, 623–634, https://doi.org/10.5194/cp-12-623-2016, 2016. a
Petera-Zganiacz, J. and Dzieduszyńska, D. A.: Palaeoenvironmental Proxies for Permafrost Presence During the Younger Dryas, Central Poland, Permafrost Periglac., 28, 726–740, 2017. a
Piotrowski, J. A.: Tunnel-valley formation in northwest Germany-geology, mechanisms of formation and subglacial bed conditions for the Bornhöved tunnel valley, Sediment. Geol., 89, 107–141, 1994. a
Piotrowski, J. A.: Subglacial groundwater flow during the last deglaciation in northwestern Germany, Sediment. Geol., 111, 217–224, 1997. a
Piotrowski, J. A.: Channelized subglacial drainage under soft-bedded ice sheets: Evidence from small N-channels in Central European Lowland, Geol. Q., 43, 153–162, 1999. a, b
Piotrowski, J. A., Geletneky, J., and Vater, R.: Soft-bedded subglacial meltwater channel from the Welzow-Sud open-cast lignite mine, Lower Lusatia, eastern Germany, Boreas, 28, 363–374, 1999. a
Queiroz Alves, E., Wong, W., Hefter, J., Grotheer, H., Tesi, T., Gentz, T., Zonneveld, K. A. F., and Mollenhauer, G.: Deglacial export of pre-aged terrigenous carbon to the Bay of Biscay, PANGAEA, https://doi.pangaea.de/10.1594/PANGAEA.954937 2023. a
Raymond, P. A., Bauer, J. E., Caraco, N. F., Cole, J. J., Longworth, B., and Petsch, S. T.: Controls on the variability of organic matter and dissolved inorganic carbon ages in northeast US rivers, Mar. Chem., 92, 353–366, 2004. a
Reimer, P. J., Austin, W. E., Bard, E., Bayliss, A., Blackwell, P. G., Bronk Ramsey, C., Butzin, M., Cheng, H., Edwards, R. L., Friedrich, M., Grootes, P. M., Guilderson, T. P., Hajdas, I., Heaton, T. J., Hogg, A. G., Hughen, K. A., Kromer, B., Manning, S. W., Muscheler, R., Palmer, J. G., Pearson, C., Van Der Plicht, J., Reimer, R. W., Richards, D. A., Scott, E. M., Southon, J. R., Turney, C. S., Wacker, L., Adolphi, F., Büntgen, U., Capano, M., Fahrni, S. M., Fogtmann-Schulz, A., Friedrich, R., Köhler, P., Kudsk, S., Miyake, F., Olsen, J., Reinig, F., Sakamoto, M., Sookdeo, A., and Talamo, S.: The IntCal20 Northern Hemisphere Radiocarbon Age Calibration Curve (0–55 cal kBP), Radiocarbon, 62, 725–757, 2020. a
Renssen, H. and Vandenberghe, J.: Investigation of the relationship between permafrost distribution in NW Europe and extensive winter sea-ice cover in the North Atlantic Ocean during the cold phases of the Last Glaciation, Quaternary Sci. Rev., 22, 209–223, 2003. a, b
Rinterknecht, V. R., Clark, P. U., Raisbeck, G. M., Yiou, F., Bitinas, A., Brook, E. J., Marks, L., Zelcs, V., Lunkka, J. P., Pavlovskaya, I. E., Piotrowski, J. A., and Raukas, A.: The Last Deglaciation of the Southeastern Sector of the Scandinavian Ice Sheet, Science, 311, 1449–1453, 2006. a, b
Rommerskirchen, F., Eglinton, G., Dupont, L., and Rullkötter, J.: Glacial/interglacial changes in southern Africa: Compoundspecific δ13C land plant biomarker and pollen records from southeast Atlantic continental margin sediments, Geochem. Geophy. Geosy., 7, 8, Q08010, https://doi.org/10.1029/2005GC001223, 2006. a
Rostek, F. and Bard, E.: Hydrological changes in eastern Europe during the last 40,000yr inferred from biomarkers in Black Sea sediments, Quaternary Res., 80, 502–509, https://doi.org/10.1016/j.yqres.2013.07.003, 2013. a, b
Schaefer, K., Lantuit, H., Romanovsky, V. E., Schuur, E. A., and Witt, R.: The impact of the permafrost carbon feedback on global climate, Environ. Res. Lett., 9, 085003, https://doi.org/10.1088/1748-9326/9/8/085003, 2014. a, b
Schefuß, E., Eglinton, T. I., Spencer-Jones, C. L., Rullkötter, J., De Pol-Holz, R., Talbot, H. M., Grootes, P. M., and Schneider, R. R.: Hydrologic control of carbon cycling and aged carbon discharge in the Congo River basin, Nat. Geosci., 9, 687–690, 2016. a, b, c
Schneider von Deimling, T., Grosse, G., Strauss, J., Schirrmeister, L., Morgenstern, A., Schaphoff, S., Meinshausen, M., and Boike, J.: Observation-based modelling of permafrost carbon fluxes with accounting for deep carbon deposits and thermokarst activity, Biogeosciences, 12, 3469–3488, https://doi.org/10.5194/bg-12-3469-2015, 2015. a, b
Schokker, J., Cleveringa, P., and Murray, A. S.: Palaeoenvironmental reconstruction and OSL dating of terrestrial Eemian deposits in the southeastern Netherlands, J. Quaternary Sci., 19, 193–202, 2004. a
Schouten, S., Hopmans, E. C., and Sinninghe Damsté, J. S.: The organic geochemistry of glycerol dialkyl glycerol tetraether lipids: A review, Org. Geochem., 54, 19–61, https://doi.org/10.1016/j.orggeochem.2012.09.006, 2013. a
Schuur, E. A., Bockheim, J., Canadell, J. G., Euskirchen, E., Field, C. B., Goryachkin, S. V., Hagemann, S., Kuhry, P., Lafleur, P. M., Lee, H., Mazhitova, G., Nelson, F. E., Rinke, A., Romanovsky, V. E., Shiklomanov, N., Tarnocai, C., Venevsky, S., Vogel, J. G., and Zimov, S. A.: Vulnerability of permafrost carbon to climate change: Implications for the global carbon cycle, BioScience, 58, 701–714, 2008. a
Schuur, E. A., Vogel, J. G., Crummer, K. G., Lee, H., Sickman, J. O., and Osterkamp, T. E.: The effect of permafrost thaw on old carbon release and net carbon exchange from tundra, Nature, 459, 556–559, https://doi.org/10.1038/nature08031, 2009. a
Schuur, E. A., McGuire, A. D., Schädel, C., Grosse, G., Harden, J. W., Hayes, D. J., Hugelius, G., Koven, C. D., Kuhry, P., Lawrence, D. M., Natali, S. M., Olefeldt, D., Romanovsky, V. E., Schaefer, K., Turetsky, M. R., Treat, C. C., and Vonk, J. E.: Climate change and the permafrost carbon feedback, Nature, 520, 171–179, 2015. a, b
Sidorchuk, A. Y., Panin, A. V., and Borisova, O. K.: Morphology of river channels and surface runoff in the Volga River basin (East European Plain) during the Late Glacial period, Geomorphology, 113, 137–157, 2009. a
Sidorchuk, A. Y., Panin, A. V., and Borisova, O. K.: Surface runoff to the Black Sea from the East European plain during Last Glacial Maximum-Late Glacial time, Special Paper of the Geological Society of America, 473, 1–25, 2011. a
Simmons, C. T., Matthews, H. D., and Mysak, L. A.: Deglacial climate, carbon cycle and ocean chemistry changes in response to a terrestrial carbon release, Clim. Dynam., 46, 1287–1299, 2016. a
Sinninghe Damsté, J. S., Hopmans, E. C., Pancost, R. D., Schouten, S., and Geenevasen, J. A.: Newly discovered non-isoprenoid glycerol dialkyl glycerol tetraether lipids in sediments, Chem. Commun., 17, 1683–1684, 2000. a
Soulet, G., Ménot, G., Bayon, G., Rostek, F., Ponzevera, E., Toucanne, S., Lericolais, G., and Bard, E.: Abrupt drainage cycles of the Fennoscandian Ice Sheet, P. Natl. Acad. Sci. USA, 110, 6682–6687, 2013. a, b
Stuiver, M. and Polach, H.: Reporting of 14C data, Radiocarbon, 19, 355–363, 1977. a
Sun, S., Meyer, V. D., Dolman, A. M., Winterfeld, M., Hefter, J., Dummann, W., McIntyre, C., Montluçon, D. B., Haghipour, N., Wacker, L., Gentz, T., Van Der Voort, T. S., Eglinton, T. I., and Mollenhauer, G.: 14C Blank Assessment in Small-Scale Compound-Specific Radiocarbon Analysis of Lipid Biomarkers and Lignin Phenols, Radiocarbon, 62, 207–218, 2020. a
Svendsen, J. I., Alexanderson, H., Astakhov, V. I., Demidov, I., Dowdeswell, J. A., Funder, S., Gataullin, V., Henriksen, M., Hjort, C., Houmark-Nielsen, M., Hubberten, H. W., Ingólfsson, Ó., Jakobsson, M., Kjær, K. H., Larsen, E., Lokrantz, H., Lunkka, J. P., Lyså, A., Mangerud, J., Matiouchkov, A., Murray, A., Möller, P., Niessen, F., Nikolskaya, O., Polyak, L., Saarnisto, M., Siegert, C., Siegert, M. J., Spielhagen, R. F., and Stein, R.: Late Quaternary ice sheet history of northern Eurasia, Quaternary Sci. Rev., 23, 1229–1271, 2004. a
Tesi, T., Muschitiello, F., Smittenberg, R. H., Jakobsson, M., Vonk, J. E., Hill, P., Andersson, A., Kirchner, N., Noormets, R., Dudarev, O., Semiletov, I., and Gustafsson: Massive remobilization of permafrost carbon during post-glacial warming, Nat. Commun., 7, 1–10, 2016. a
Toucanne, S., Zaragosi, S., Bourillet, J. F., Cremer, M., Eynaud, F., Van Vliet-Lanoë, B., Penaud, A., Fontanier, C., Turon, J. L., Cortijo, E., and Gibbard, P. L.: Timing of massive “Fleuve Manche” discharges over the last 350 kyr: insights into the European ice-sheet oscillations and the European drainage network from MIS 10 to 2, Quaternary Sci. Rev., 28, 1238–1256, https://doi.org/10.1016/j.quascirev.2009.01.006, 2009. a, b
Toucanne, S., Zaragosi, S., Bourillet, J. F., Marieu, V., Cremer, M., Kageyama, M., Van Vliet-Lanoë, B., Eynaud, F., Turon, J. L., and Gibbard, P. L.: The first estimation of Fleuve Manche palaeoriver discharge during the last deglaciation: Evidence for Fennoscandian ice sheet meltwater flow in the English Channel ca 20–18 ka ago, Earth Planet. Sc. Lett., 290, 459–473, https://doi.org/10.1016/j.epsl.2009.12.050, 2010. a, b, c
Toucanne, S., Soulet, G., Freslon, N., Silva Jacinto, R., Dennielou, B., Zaragosi, S., Eynaud, F., Bourillet, J. F., and Bayon, G.: Millennial-scale fluctuations of the European Ice Sheet at the end of the last glacial, and their potential impact on global climate, Quaternary Sci. Rev., 123, 113–133, 2015. a, b, c, d
Treat, C. C., Kleinen, T., Broothaerts, N., Dalton, A. S., Dommaine, R., Douglas, T. A., Drexler, J. Z., Finkelstein, S. A., Grosse, G., Hope, G., Hutchings, J., Jones, M. C., Kuhry, P., Lacourse, T., Lähteenoja, O., Loisel, J., Notebaert, B., Payne, R. J., Peteet, D. M., Sannel, A. B. K., Stelling, J. M., Strauss, J., Swindles, G. T., Talbot, J., Tarnocai, C., Verstraeten, G., Williams, C. J., Xia, Z., Yu, Z., Väliranta, M., Hättestrand, M., Alexanderson, H., and Brovkin, V.: Widespread global peatland establishment and persistence over the last 130,000 y, P. Natl. Acad. Sci. USA, 116, 4822–4827, 2019. a, b
Turner, C.: The Eemian interglacial in the North European plain and adjacent areas, Geol. Mijnbouw-N. J. G., 79, 217–231, 2000. a
Van Huissteden, J., Vandenberghe, J., Van Der Hammen, T., and Laan, W.: Fluvial and aeolian interaction under permafrost conditions: Weichselian Late Pleniglacial, Twente, eastern Netherlands, Catena, 40, 307–321, 2000. a
Vandenberghe, J. and Pissart, A.: Permafrost changes in Europe during the last glacial, Permafrost Periglac., 4, 121–135, 1993. a, b, c, d
Vandenberghe, J., Renssen, H., Roche, D. M., Goosse, H., Velichko, A. A., Gorbunov, A., and Levavasseur, G.: Eurasian permafrost instability constrained by reduced sea-ice cover, Quaternary Sci. Rev., 34, 16–23, 2012. a, b
Vandenberghe, J., French, H. M., Gorbunov, A., Marchenko, S., Velichko, A. A., Jin, H., Cui, Z., Zhang, T., and Wan, X.: The Last Permafrost Maximum (LPM) map of the Northern Hemisphere: Permafrost extent and mean annual air temperatures, 25–17 ka BP, Boreas, 43, 652–666, 2014. a, b, c
Vonk, J. E., Sanchez-Garca, L., Van Dongen, B. E., Alling, V., Kosmach, D., Charkin, A., Semiletov, I. P., Dudarev, O. V., Shakhova, N., Roos, P., Eglinton, T. I., Andersson, A., and Gustafsson, A.: Activation of old carbon by erosion of coastal and subsea permafrost in Arctic Siberia, Nature, 489, 137–140, 2012. a
Wacker, L., Christl, M., and Synal, H. A.: Bats: A new tool for AMS data reduction, Nuclear Instrum. Meth. B, 268, 976–979, 2010. a
Wainer, K., Genty, D., Blamart, D., Daëron, M., Bar-Matthews, M., Vonhof, H., Dublyansky, Y., Pons-Branchu, E., Thomas, L., van Calsteren, P., Quinif, Y., and Caillon, N.: Speleothem record of the last 180 ka in Villars cave (SW France): Investigation of a large δ18O shift between MIS6 and MIS5, Quaternary Sci. Rev., 30, 130–146, 2011. a, b
Wang, M. J., Zheng, H. B., Xie, X., Fan, D. D., Yang, S. Y., Zhao, Q. H., and Wang, K.: A 600-year flood history in the Yangtze River drainage: Comparison between a subaqueous delta and historical records, Chinese Sci. Bull., 56, 188–195, 2011. a, b
Weltje, G. J. and Tjallingii, R.: Calibration of XRF core scanners for quantitative geochemical logging of sediment cores: Theory and application, Earth Planet. Sc. Lett., 274, 423–438, 2008. a
Winterfeld, M., Mollenhauer, G., Dummann, W., Köhler, P., Lembke-Jene, L., Meyer, V. D., Hefter, J., McIntyre, C., Wacker, L., Kokfelt, U., and Tiedemann, R.: Deglacial mobilization of pre-aged terrestrial carbon from degrading permafrost, Nat. Commun., 9, 3666, https://doi.org/10.1038/s41467-018-06080-w, 2018. a, b, c, d, e
Woronko, B., Rychel, J., Karasiewicz, T. M., Kupryjanowicz, M., Adamczyk, A., Fiłoc, M., Marks, L., Krzywicki, T., and Pochocka-Szwarc, K.: Post-Saalian transformation of dry valleys in eastern Europe: An example from NE Poland, Quatern. Int., 467, 161–177, 2018. a
Wu, J., Mollenhauer, G., Stein, R., Köhler, P., Hefter, J., Fahl, K., Grotheer, H., Wei, B., and Nam, S.-I.: Deglacial release of petrogenic and permafrost carbon from the Canadian Arctic impacting the carbon cycle, Nat. Commun., 13, 1–11, 2022. a, b, c
Wu, L., Wilson, D. J., Wang, R., Yin, X., Chen, Z., Xiao, W., and Huang, M.: Evaluating Zr Rb Ratio From XRF Scanning as an Indicator of Grain-Size Variations of Glaciomarine Sediments in the Southern Ocean, Geochem. Geophy. Geosy., 21, e2020GC009350, https://doi.org/10.1029/2020GC009350, 2020. a
Žák, K., Richter, D. K., Filippi, M., Živor, R., Deininger, M., Mangini, A., and Scholz, D.: Coarsely crystalline cryogenic cave carbonate – a new archive to estimate the Last Glacial minimum permafrost depth in Central Europe, Clim. Past, 8, 1821–1837, https://doi.org/10.5194/cp-8-1821-2012, 2012. a
Zhang, Y., Chiessi, C. M., Mulitza, S., Zabel, M., Trindade, R. I., Hollanda, M. H. B., Dantas, E. L., Govin, A., Tiedemann, R., and Wefer, G.: Origin of increased terrigenous supply to the NE South American continental margin during Heinrich Stadial 1 and the Younger Dryas, Earth Planet. Sc. Lett., 432, 493–500, 2015. a
Zhao, Z., Ahlberg, P., Thibault, N., Dahl, T. W., Schovsbo, N. H., and Nielsen, A. T.: High-resolution carbon isotope chemostratigraphy of the middle Cambrian to lowermost Ordovician in southern Scandinavia: Implications for global correlation, Global Planet. Change, 209, 103751, https://doi.org/10.1016/j.gloplacha.2022.103751, 2022. a, b
Zhou, B., Zheng, H., Yang, W., Taylor, D., Lu, Y., Wei, G., Li, L., and Wang, H.: Climate and vegetation variations since the LGM recorded by biomarkers from a sediment core in the northern South China Sea, J. Quaternary Sci., 27, 948–955, 2012. a
Zimov, S. A., Davydov, S. P., Zimova, G. M., Davydova, A. I., Schuur, E. A., Dutta, K., and Chapin, I. S.: Permafrost carbon: Stock and decomposability of a globally significant carbon pool, Geophys. Res. Lett., 33, L20502, https://doi.org/10.1029/2006GL027484, 2006. a