the Creative Commons Attribution 4.0 License.
the Creative Commons Attribution 4.0 License.
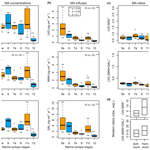
Relationships between low-temperature fires, climate and vegetation during three late glacials and interglacials of the last 430 kyr in northeastern Siberia reconstructed from monosaccharide anhydrides in Lake El'gygytgyn sediments
Kai Mangelsdorf
Andrei Andreev
Cornelia Karger
Laura T. Schreuder
Ellen C. Hopmans
Oliver Rach
Dirk Sachse
Volker Wennrich
Ulrike Herzschuh
Landscapes in high northern latitudes are assumed to be highly sensitive to future global change, but the rates and long-term trajectories of changes are rather uncertain. In the boreal zone, fires are an important factor in climate–vegetation interactions and biogeochemical cycles. Fire regimes are characterized by small, frequent, low-intensity fires within summergreen boreal forests dominated by larch, whereas evergreen boreal forests dominated by spruce and pine burn large areas less frequently but at higher intensities. Here, we explore the potential of the monosaccharide anhydrides (MA) levoglucosan, mannosan and galactosan to serve as proxies of low-intensity biomass burning in glacial-to-interglacial lake sediments from the high northern latitudes. We use sediments from Lake El'gygytgyn (cores PG 1351 and ICDP 5011-1), located in the far north-east of Russia, and study glacial and interglacial samples of the last 430 kyr (marine isotope stages 5e, 6, 7e, 8, 11c and 12) that had different climate and biome configurations. Combined with pollen and non-pollen palynomorph records from the same samples, we assess how far the modern relationships between fire, climate and vegetation persisted during the past, on orbital to centennial timescales. We find that MAs attached to particulates were well-preserved in up to 430 kyr old sediments with higher influxes from low-intensity biomass burning in interglacials compared to glacials. MA influxes significantly increase when summergreen boreal forest spreads closer to the lake, whereas they decrease when tundra-steppe environments and, especially, Sphagnum peatlands spread. This suggests that low-temperature fires are a typical characteristic of Siberian larch forests also on long timescales. The results also suggest that low-intensity fires would be reduced by vegetation shifts towards very dry environments due to reduced biomass availability, as well as by shifts towards peatlands, which limits fuel dryness. In addition, we observed very low MA ratios, which we interpret as high contributions of galactosan and mannosan from biomass sources other than those currently monitored, such as the moss–lichen mats in the understorey of the summergreen boreal forest. Overall, sedimentary MAs can provide a powerful proxy for fire regime reconstructions and extend our knowledge of long-term natural fire–climate–vegetation feedbacks in the high northern latitudes.
- Article
(4290 KB) - Full-text XML
- BibTeX
- EndNote
Background consideration
In recent decades, high northern latitudes have experienced a warming at more than twice the rate of other regions on Earth (Serreze and Barry, 2011; IPCC, 2014). This Arctic amplification has widespread impacts on the Earth system, such as the hydrological cycle and carbon budgets (Schuur et al., 2015; Linderholm et al., 2018). The likelihood of wildfire is increasing due to warmer temperatures, more lightening-induced ignition and potential shifts towards more flammable vegetation (Soja et al., 2007; Hu et al., 2010; Tautenhahn et al., 2016; Veraverbeke et al., 2017; Nitze et al., 2018). However, the rates and directions of fire regime and vegetation change are poorly constrained (Abbott et al., 2016).
Climate and vegetation types shape regional fire regimes (characterized by fire frequency, intensity, severity, seasonality, area and type and amount of biomass burned; Harris et al., 2016). Climate influences biomass availability and flammability via the length and conditions of the growing season, the type of biomass available to burn, and weather conditions that affect local soil conditions and fuel dryness affecting fire spread (Westerling et al., 2006). Accordingly, biomass burning is related to temperature variability on centennial to orbital timescales (Daniau et al., 2012; Marlon et al., 2013).
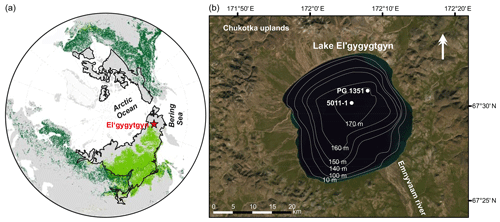
Figure 1(a) Distribution of high-northern summer- and evergreen boreal forest (light and dark green, respectively) and location of Lake El'gygytgyn (red star). Black lines mark approximate modern continuous permafrost extent (after Williams and Ferrigno, 2012), land cover classification based on data © ESA Climate Change Initiative Land cover project, and land cover CCI, provided via the Centre for Environmental Data Archival (CEDA). (b) Lake El'gygytgyn and location of analysed sediment cores (map: © Landsat-7 image, courtesy of the U.S. Geological Survey).
Vegetation drives fires by developing individual plant traits, which determine flammability and regeneration strategies (Rogers et al., 2015; Feurdean et al., 2020) and lead to tight internal fire–vegetation feedbacks (Krawchuk and Moritz, 2011; Pausas et al., 2017). Modern fire regimes differ strongly in the high-northern biomes (Fig. 1), such as between tundra and Siberian summergreen and evergreen boreal forests (Wirth, 2005; Sofronov and Volokitina, 2010; Rogers et al., 2015), with less than 1 % of Arctic and subarctic tundra being affected by fires compared to ca. 8 % of eastern Siberian boreal forest (Nitze et al., 2018).
In the tundra, grasses and shrubs burn rarely and at low fire intensities, due to soil and organic matter drying-up during the short summer seasons and limited fuel availability (Krawchuk and Moritz, 2011). While a single fire has several phases of varying fire intensities, fire regimes define the larger temporal and spatial scale properties of several fire events. Regimes of low fire intensity generally refer to low fire temperatures, low combustion efficiencies and low fire radiative power as is typical for smoldering and in contrast to flaming fires (Keeley, 2009; Conedera et al., 2009; van Leeuwen and van der Werf, 2011). In Siberian summergreen boreal forests, dominated by larch (mainly Larix gmelenii and L. cajanderi) and shrub alder (Alnus fruticosa) (Isaev et al., 2010), frequent low-intensity surface fires burn the understorey during dry summers (Kharuk et al., 2011). These fires characterize a “pyrome” of rare, cool and small events (Archibald et al., 2013) with fires that are mainly non-stand-replacing and of incomplete combustion (Rogers et al., 2015; van der Werf et al., 2017; Chen and Loboda, 2018). Fire is suspected to support the existence and regeneration of larch by, for example, selectively reducing regrowth and within-species competition (Kharuk et al., 2011; Zhang et al., 2011; Tautenhahn et al., 2016). In addition, larch is a fire resister with its fire-protecting bark and shedding of the deciduous foliage that limits fire spreading to the crowns (Wirth, 2005). In contrast, Siberian evergreen boreal forests are dominated by Siberian pine (Pinus sibirica), spruce (Picea obovata) and fir (Abies sibirica), which are fire avoiders that rarely burn and hardly regenerate after fire and that suppress fires due to a rather wet understorey, whereas Pinus sylvestris stands are highly flammable and can resist infrequent fires (Furyaev et al., 2001; Wirth, 2005; Isaev et al., 2010; Rogers et al., 2015; Tautenhahn et al., 2016). Hence, wildfires are rare, but once ignited they burn large areas at high intensities (Archibald et al., 2013) because fires can spread quickly to the crowns via the resin-rich conifer needles (Rogers et al., 2015; van der Werf et al., 2017).
While it is well-recognized that alternative stable states of biome configuration can be driven by fire (Lasslop et al., 2016) and can characterize the boreal forest biomes (Scheffer et al., 2012; Rogers et al., 2015), the causes, feedbacks and thresholds that lead to shifts between stable states are still debated (Tchebakova et al., 2009; Gonzalez et al., 2010; Loranty et al., 2014; Abbott et al., 2016). Recently, Herzschuh et al. (2016) proposed that fire plays an important role in long-term climate–vegetation interactions and internal system feedbacks that determine alternative stable states in high northern biomes. However, knowledge of past fire regimes and associated natural feedbacks in the high latitudes on long centennial to orbital timescales is scarce. Previous interglacials provide analogues for a warming world (Yin and Berger, 2015) beyond human influence in contrast to the Holocene, when lightning was not the only source of ignition (Buchholz et al., 2003; Marlon et al., 2013; Veraverbeke et al., 2017; Dietze et al., 2018).
Lake El'gygytgyn sediment cores provide a continuous Pliocene–Pleistocene environmental record in the Arctic reflecting strong climate and vegetation shifts during the past 3.6 Myr (Melles et al., 2012; Brigham-Grette et al., 2013; Tarasov et al., 2013; Andreev et al., 2014, 2016; Wennrich et al., 2016). Lake El'gygytgyn is a meteorite impact crater lake of 110 km2, a diameter of ∼12 km and maximum water depth of 170 m formed about 3.6 Myr ago (Wennrich et al., 2016, and references therein). The 183 km2 large catchment and wider region of NE Siberia is dominated by volcanic and metamorphic rocks and permanently frozen Quaternary deposits below an active layer of up to 80 cm depth (Schwamborn et al., 2006). Climate conditions are cold, dry and windy, with a mean annual air temperature of ca. −10 ∘C and an annual precipitation of ca. 180 mm (in 2002), mainly falling as snow (Nolan and Brigham-Grette, 2007). Current treeless herb tundra vegetation is composed of patches of lichens and herbs (mostly Poaceae and Cyperaceae) next to barren ground and a few dispersed dwarf shrubs of Salix and Betula near the lake and of Pinus pumila and Alnus in the surrounding Chukchi Uplands (Lozhkin et al., 2007). The treeline towards the summergreen boreal forest is located ca. 100–150 km to the south-west (Fig. 1a).
To reconstruct long-term fire regime shifts, sedimentary charcoal can be used as a classical proxy for fire of various combustion efficiencies (Whitlock and Larsen, 2001; Conedera et al., 2009). Yet, fire intensity reconstructions and the differentiation between surface and crown fires is difficult to specify, especially on long timescales, as well as when considering other fire proxies such as fire scars and fungal spores (Stivrins et al., 2019). Molecular burning proxies are currently being explored to infer source- and temperature-specific fire histories (Han et al., 2016; Kappenberg et al., 2019; Dietze et al., 2019). Unique proxies of biomass burning from low-intensity fires are the monosaccharide anhydrides (MAs) levoglucosan (1,6-anhydro-β-D-glucopyranose, LVG) and its isomers mannosan (1,6-anhydro-β-D-mannopyranose, MAN) and galactosan (1,6-anhydro-β-D-galactopyranose, GAL). While Simoneit et al. (1999) and references therein suggest that MAs form at burn temperatures > 300 ∘C, several studies that have analysed the influence of various combustion conditions in natural samples indicated that MAs are thermal dehydration products at burning temperatures < 350 ∘C, mainly under smouldering as opposed to flaming conditions (Pastorova et al., 1993; Gao et al., 2003; Engling et al., 2006; Kuo et al., 2008, 2011). A recent review of Suciu et al. (2019) attributes the dominant low-temperature production to depolymerization, fragmentation and inter- and intramolecular transglycosylation during pyrolysis, while a minor fraction of MAs can attach to charcoal during higher temperatures, when smoldering overlaps with or follows flaming conditions. In Holocene lake sediments, MAs provide complementary fire proxies to sedimentary charcoal (Elias et al., 2001; Schüpbach et al., 2015; Battistel et al., 2017; Schreuder et al., 2019; Dietze et al., 2019). While LVG is preserved in marine sediment for at least the last 130 kyr (Lopes dos Santos et al., 2013), to our knowledge, sedimentary MAs not been analysed in either high-latitude or in interglacial lake sediments.
Here, we assess (1) if MAs are useful proxies to study glacial-to-interglacial fire histories during the last 430 kyr using sedimentary MA from Lake El'gygytgyn and (2) discuss long-term relationships between low-temperature fires and regional vegetation in the Russian Far East (Fig. 1). We selected three late glacial-to-interglacial periods, i.e. marine isotope stages (MISs) 12–11c, 8–7e and 6–5e, which reflect varying interglacial biome types and climate conditions, as reconstructed using the pollen records from El'gygytgyn sediments (Melles et al., 2012; Tarasov et al., 2013). The so-called “superinterglacial” MIS 11c (ca. 420–380 kyr ago) has been described as warmer and wetter compared to today, which was supported by biomarker-based temperature reconstructions (D'Anjou et al., 2013). The presence of spruce (Picea) pollen in El'gygytgyn sediments suggests that the evergreen boreal forest has been much closer to the lake than today (Melles et al., 2012; Tarasov et al., 2013; Lozhkin et al., 2017). In contrast, MIS 7e (ca. 240–220 kyr ago) was cooler than today, and only a few coniferous pollen grains were found. Birch and alder pollen suggest that shrub tundra prevailed during this interglacial (Lozhkin et al., 2007; Zhao et al., 2019). MIS 5e interglacial (ca. 130–110 kyr ago) was slightly warmer than today (Tarasov et al., 2013). Larch and alder pollen found in El'gygytgyn sediments suggest that a summergreen boreal forest existed close to the lake, whereas spruce pollen was absent (Lozhkin et al., 2007). These differences in regional vegetation and climate conditions have been explained by changes in global ice volume, insolation (for interglacial astronomical and greenhouse gas concentrations characteristics see Yin and Berger, 2012, and their Table 1) and interhemispheric ice sheet–ocean–atmosphere feedback mechanisms (Melles et al., 2012; Lozhkin et al., 2017).
Here, we aim to answer (1) whether sedimentary MAs are suitable proxies to reconstruct low-temperature fires even in interglacial Arctic lake sediments and (2) whether more biomass burning occurs during interglacials compared to late glacials due to climate-driven changes in biomass availability, since wildfires are expected to be fuel-limited in cold environments (Krawchuk and Moritz, 2011; Daniau et al., 2012). Furthermore, as different vegetation composition has been reconstructed from past interglacials (Melles et al., 2012; Brigham-Grette et al., 2013; Andreev et al., 2014), we consider (3) whether more low-temperature biomass burning occurs in summergreen boreal forest compared to tundra or evergreen boreal forest during interglacials on centennial to millennial timescales.
2.1 Sample selection and preparation
We sampled sediment from two cores (Fig. 1b). A 12.7 m long sediment core – PG1351 – was recovered in 1998 and covers the last 270 kyr, according to 14C- and luminescence dates, as well as magnetostratigraphy (Nowaczyk et al., 2007, 2013; Melles et al., 2007). A 318 m long composite core from ICDP site 5011-1 comprises three parallel sediment cores that cover the time period 125 to 3600 kyr (Melles et al., 2011; Wennrich et al., 2016), based on magnetostratigraphy and orbital tuning with more than 600 chronologic tie points (Nowaczyk et al., 2013).
For MA analyses, we freeze-dried and homogenized 44 samples of ca. 0.7–1.8 g dry sediment from core PG1351 covering late glacials and interglacials of MIS 8 to MIS 5e, integrating sediment of 1 cm core depth. Temporal resolution of these samples ranges from 140 to 960 years per sample. For the period between 430 and 405 kyr ago (end of MIS 12 to MIS 11c), 13 samples of 0.5–1.3 g of dry sediment from ICDP core 5011-1 were taken for MA analyses, integrating sediment of 2 cm core depth. Eight of these 13 samples are from the same core depths as were previously analysed for pollen (Melles et al., 2012). Temporal resolution of these samples varies between 200 and 970 years per sample, comparable to core PG1351. Across all samples, temporal resolution is 333±273 years per sample, giving centennial- to millennial-scale averages.
We extracted the polar lipids of all MA samples using a Dionex accelerated solvent extraction system (ASE 350, Thermo Fisher Scientific) at 100 ∘C, 103 bar pressure and two extraction cycles (20 min static time) with 100 % methanol, after an ASE cycle with 100 % dichloromethane. For every sample sequence (n=13–18), we extracted a blank ASE cell and included it in all further steps. We added 60 ng of deuterated levoglucosan (dLVG; C6H3D7O5; Th. Geyer GmbH & Co. KG) as internal standard and filtered the extract over a PTFE filter using acetonitrile and 5 % HPLC-grade water. We analysed the extracts with an Ultimate 3000 RS ultra-high-performance liquid chromatograph (U-HPLC) with thermostated autosampler and column oven coupled to a Q Exactive Plus Orbitrap mass spectrometer (Quadrupole-Orbitrap MS; Thermo Fisher Scientific) with heated electrospray injection (HESI) probe at GFZ Potsdam, using measurement conditions adapted from earlier studies (Hopmans et al., 2013; Schreuder et al., 2018; Dietze et al., 2019). Briefly, separation was achieved on two Xbridge BEH amide columns in series (2.1×150 mm, 3.5 µm particle size) fitted with a 50 mm precolumn of the same material (Waters). The compounds were eluted (flow rate of 0.2 mL min−1) with 100 % A for 15 min, followed by column cleaning with 100 % B for 15 min and re-equilibration to starting conditions for 25 min. Eluent A was acetonitrile : water : triethylamine () and eluent B acetonitrile : water : triethylamine (). HESI settings were as follows: sheath gas (N2) pressure of 20 (arbitrary units), auxiliary gas (N2) pressure of 3 (arbitrary units), auxiliary gas (N2) temperature of 50 ∘C, spray voltage of −2.9 kV (negative ion mode), capillary temperature of 300 ∘C and S-Lens of 50 V. Detection was achieved by monitoring m∕z 150–200 with a resolution of 280 000 ppm. Targeted data-dependent MS2 (normalized collision energy 13 V) was performed on any signal within 10 ppm of m∕z 161.0445 (calculated exact mass of deprotonated levoglucosan and its isomers) or m∕z 168.0884 (calculated exact mass of deprotonated dLVG) with an isolation window of 0.4 m∕z. The detection limit was 2.5 pg on column, based on injections of 0.5 to 5000 pg on column of authentic standards of LVG, MAN and GAL (Santa Cruz Biotechnology) and dLVG.
Integrations were performed on mass chromatograms within 3 ppm mass accuracy and corrected for relative response factors to dLVG (1.08±0.10, 0.76±0.10 and 0.24±0.05 for LVG, MAN and GAL, respectively), according to known authentic standard mixes injected before and after every measurement sequence and supported by characteristic isomer-specific MS2 data. All samples were corrected by subtracting the maximum MA concentrations in the blank duplicates of each ASE sequence. To account for biases due to sediment properties and sedimentation rates, MA influxes (mass accumulation rates, ng cm−2 yr−1) were calculated by multiplying the concentrations (ng g−1) with the sample-specific dry bulk densities (Melles et al., 2007; Wennrich et al., 2016) and the sedimentation rates of the sample (cm yr−1) using the age–depth models presented by Nowaczyk et al. (2013) for the PG1351 and the ICDP-5011-1 cores.
Pollen from sediment core PG1351 was analysed by Lozhkin et al. (2007), but there was no sediment left to sample the same core depths for MA analyses. Therefore, we sampled 19 of the 44 MA core depths from the sample for parallel pollen analyses to enable a direct comparison of MA and pollen records without age bias. These new pollen samples were prepared using standard pollen preparation procedures as have been used previously for the El'gygytgyn sediments (Andreev et al., 2012). In addition to pollen and spores, non-pollen-palynomorphs (NPPs) such as algae remains and coprophilous fungi spores were counted. Existing pollen data (Melles et al., 2012) from the same depths as MA samples of late MIS 12 and 11c were harmonized with the new pollen samples of core PG1351; i.e. pollen types were aggregated to the highest taxonomic level identified by the two palynologists, to compare the same taxa in percentages.
2.2 Analyses of source areas
MAs can be transported attached to aerosols in the atmosphere (Sang et al., 2016; Schreuder et al., 2018) and/or via fluvial transport from the catchment (Suciu et al., 2019). To discuss potential source areas of aeolian-derived MAs, we calculated exemplary backward trajectory ensembles of 2 d towards Lake El'gygytgyn using the Hybrid Single-Particle Lagrangian Integrated Trajectory (HYSPLIT) model (Stein et al., 2015; Rolph et al., 2017) during the main boreal and tundra fire season of the summers 2017 and 2018 (May to September; http://ready.arl.noaa.gov/hypub-bin/trajtype.pl?runtype=archive, last access: 18 June 2019). Trajectories considered a mean particle injection height during a wildfire of between 100 and 1000 m after Peters and Higuera (2007).
2.3 Evaluation of relationships
MA influxes and ratios were correlated with the six most indicative pollen and NPP types excluding samples with < 0.5 % of a certain taxon to stabilize the signal-to-noise ratio (Prentice et al., 1996). Evergreen boreal forest is represented by Picea pollen and summergreen boreal forest (SGB) by the sum of Larix, Alnus and Populus pollen percentages. The sum of Poaceae, Artemisia, Chenopodiaceae, Caryophyllaceae, Cichoriaceae and Thalictrum pollen represent typical taxa of cold tundra-steppe environments. Pinus s/g. Haploxylon-type pollen can be produced by a shrub stone pine (Pinus pumila), which does not survive fires, or by P. sibirica, a Siberian pine that can survive but is not adapted to fires (Ma et al., 2008; Keeley, 2012). Sphagnum spores reflect wet habitats (peatlands), whereas Selaginella rupestris spores from a ledge or rock spike-moss are indicative of extremely dry and cold habitats.
To test whether mean MA influxes of interglacial and their preceding late glacial sediments were equal (null hypothesis) or significantly higher or lower, we performed the nonparametric Wilcoxon rank sum test with determination of an exact permutation null distribution, suited for few numbers of samples. The test statistics and associated p values were calculated using the function wilcox.test of the stats-package in R (Bauer, 1972). To quantify the relationships between low-temperature fires and vegetation, we log-transformed the MA ratios and pollen percentages to correct for their skewed distributions. Then, we correlated MA records with the major land cover types using Kendall's τ rank correlation coefficient, which is robust against outliers, small sample sizes and spurious correlations, in contrast to Pearson's correlation coefficient (Aitchison, 1986; Jackson and Somers, 1991; Arndt et al., 1999). Kendall's τ and associated p values were calculated using the function corr.test of the R package psych (Revelle, 2018) using pairwise complete observations. Kendall's τ is only provided for n > 5. Please note that the reported categorization of p values in Figs. 2 and 4 does not mean that some distribution differences and relationships are more valuable or “correct” than others (Wasserstein et al., 2019), as uncertainties in the data are not restricted to statistics alone (see Sect. 4.1). Full p values and test statistics are reported (see legend in top panel of Fig. 2b).
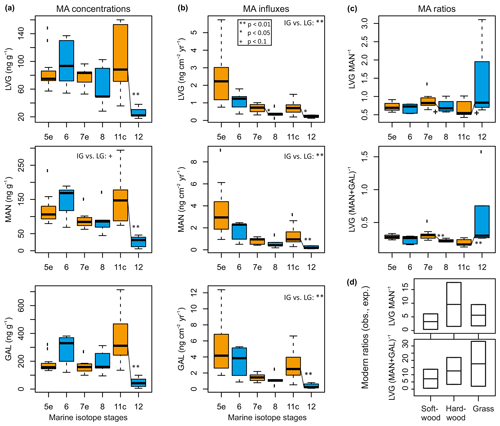
Figure 2Ranges of monosaccharide anhydride (MA) composition during selected marine isotope stages (MIS) at an orbital timescale: (a) levoglucosan (LVG), mannosan (MAN) and galactosan (GAL) concentrations; (b) influxes and (c) ratios of the three isomers. Boxplots show median, interquartile ranges (IQR: box), 1.5× the IQR (whisker) and extreme outliers (ticks outside of whiskers) of samples that cover different time spans; MIS 12: 430–424 kyr (n=5); 11c: 422–406 kyr (n=8); 8: 256–246 kyr (n=5); 7e: 242–232 kyr (n=16); 6: 145–134 kyr (n=5); and 5e: 132–117 kyr (n=18), with blue (orange) boxes marking late glacial (interglacial) periods. (d) Modern MA ratios from observations in aerosol and experimental burning after Fabbri et al. (2009), for comparison with (c). Lines between interglacial and preceding late glacial boxplots indicate different mean values for the two periods according to a nonparametric Wilcoxon test, with stars and + indicating respective p values (see legend in top panel of b).
MAs are detected well in all samples with LVG, MAN and GAL concentrations of 77±35, 109±58 and 204±129 ng g−1 (mean ± standard deviation, Fig. 2a), respectively. The standard instrumental errors from duplicate measurements are 4.9±2.9, 4.8±4.1 and 7.1±5.2 % for LVG, MAN and GAL, respectively. Blanks contained 7.6±4.7, 2.1±0.7 and 2.1±0.6 % of the respective mean LVG, MAN and GAL concentrations in the samples, derived from carryover within the ASE preparation step, and are subtracted from the concentrations of the respective sample batch.
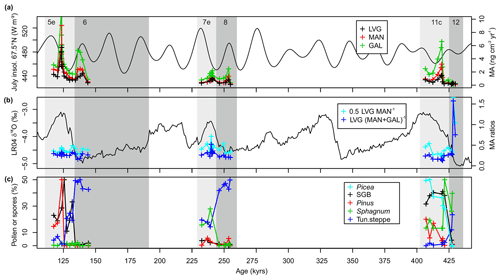
Figure 3Records of low-intensity fires and vegetation at a centennial timescale. (a) MA influxes against summer insolation (after Laskar et al., 2004); (b) MA ratios against a proxy for past ice-sheet extent (after Lisiecki and Raymo, 2005); and (c) selected pollen records (MIS 6–5e, 8–7e: this study; MIS 12–11c: after Melles et al., 2012). Tun.steppe (i.e. tundra and steppe taxa reflecting cold and dry glacial conditions): sum of Poaceae, Artemisia, Chenopodiaceae, Caryophyllaceae, Cichoriaceae and Thalictrum pollen; summergreen boreal forest taxa (SGB): sum of Larix, Populus, and Alnus pollen; Pinus s/g. Haploxylon-type pollen (with SGB and Pinus spreading during interglacials); and the Sphagnum spore abundance (representative for peatlands).
Concentrations vary during interglacials and late glacial stages, with the lowest values during MIS 12, although concentrations are strongly affected by the sediment bulk density and sedimentation rate (Fig. 2a vs. b). Hence, we focus here on relative changes in MA influxes, which are consistently higher during interglacials compared to the latter part of their preceding glacials, according to the boxplots and Wilcoxon rank sum test statistics (Fig. 2b, the Supplement). Among interglacials, influxes are highest during MIS 5e (e.g. LVGmedian: 2.2; GALmedian: 4.2 ng cm−2 yr−1) and lowest during MIS 7e (LVGmedian: 0.8; GALmedian: 1.5 ng cm−2 yr−1; Figs. 2b, 3). Highest late glacial MA influxes are found in MIS 6 samples (LVGmedian: 1.2; GALmedian: 3.8 ng cm−2 yr−1), whereas MIS 12 samples have the lowest MA influxes (LVGmedian: 0.2; GALmedian: 0.2 ng cm−2 yr−1, Fig. 2b). MA records reach their highest values during the peak of interglacials, with secondary maxima during MIS 8 and MIS 6 at times of high summer insolation (Fig. 3). MA influx records are strongly positively correlated across all intervals (LVG vs. MAN or GAL: Kendall's τ=0.76–0.82, p=0.00–0.05; MAN vs. GAL: τ=0.91–0.97, p < 0.001; Fig. 4a) with slightly closer relationships during MIS 8–5e compared to MIS 12–11c.
MA influx records are not correlated with MA ratios (Fig. 4a) except for in MIS 12–11c samples, which show a significant inverse relationship for MAN and GAL influxes with LVG (MAN+GAL)−1 (Kendall's and −0.77, p=0.05 and 0.02). Fires that produced more MAN and GAL in MIS 11c have a low isomer ratio, whereas MIS 12 samples have very low amounts of all three isomers, yet with relatively high MA ratios. MA ratios do not show consistent differences between interglacials and late glacial stages, with highest average ratios during MIS 12 and 7e, with LVG MAN: 0.82 and 0.83; LVG (MAN+GAL): 0.31 and 0.30, and lowest ratios in MIS 11c samples, LVG MAN: 0.54; LVG (MAN+GAL): 0.17. Only in a single late-MIS 12 sample do LVG MAN−1 and LVG (MAN+GAL)−1 exceed 3 and 1, respectively.
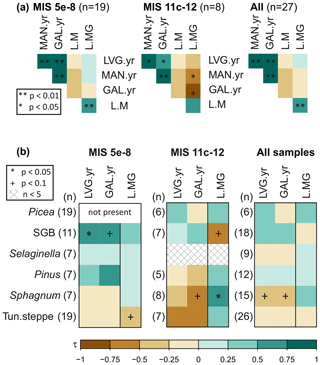
Figure 4Kendall's τ rank correlation coefficients between (a) MA influxes (LVG.yr, MAN.yr, GAL.yr) and ratios, L.M = LVG MAN−1 and L.MG = LVG (MAN+GAL)−1, and (b) selected influx and ratio record and selected pollen records. SGB: pollen sum of summergreen boreal forest taxa; Tun.steppe: sum of indicative taxa for (typical glacial) tundra-steppe environment; for further taxa see text and Fig. 3.
Vegetation compositions as reflected by the pollen and NPP records vary not only between the interglacials and their preceding late glacials but also among interglacials (Figs. 3, 5), as stated in previous studies (Lozhkin et al., 2007; Melles et al., 2012). The high proportions of Sphagnum spores during MIS 12–11c and MIS 7e suggest more widespread peatlands in contrast to MIS 8 and 6–5e. The presence of spruce during MIS 11c also indicates much warmer conditions compared to MIS 7e and 5e (Lozhkin et al., 2007; Melles et al., 2012), whereas MIS 7e is the coolest interglacial considered here, as indicated by the low amount of typical summergreen boreal and high amount of tundra steppe taxa pollen (Figs. 3, 5).
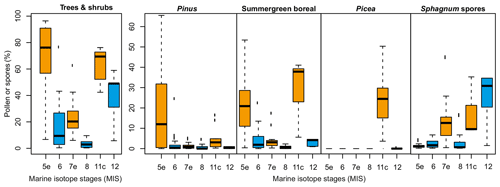
Figure 5Boxplots of vegetation composition for the marine isotope stages of interest based on pollen samples. MIS 6–5e, 8–7e: this study; MIS 12–11c: after Melles et al. (2012); SGB: sum of Larix, Populus and Alnus pollen; Pinus: P. s/g. Haploxylon-type pollen; trees and shrubs: sum of Betula SGB, Salix, Pinus and Picea; MIS 12: 430–424 kyr (n=4); MIS 11c: 423–406 kyr (n=10); MIS 8: 272–243 kyr (n=11); MIS 7e: 242–193 kyr (n=21); MIS 6: 190– 134 kyr (n=37); and MIS 5e: 132–110 kyr (n=25), with blue (orange) boxes marking late glacial (interglacial) periods.
Despite few parallel samples with n > 4 and pollen and spore amounts higher than 0.5 %, some linkages between MA and pollen/NPP records using Kendall's τ are robust, especially when considering the periods MIS 12–11c and MIS 8–5e separately (Fig. 4b). MA influxes are positively related to the summergreen boreal taxa during MIS 5e to 8 (e.g. LVG vs. log of SGB: τ=0.7, p=0.02) and showed a tendency towards positive association between GAL and pine (τ=0.6, p=0.14). During late MIS 12 and MIS 11c, we find a weak negative relationship between GAL and Sphagnum (, p=0.09) and a tendency towards lower GAL influxes during periods of widespread tundra steppe (e.g. GAL vs. tun.steppe: , p=0.14).
As both MA ratios show similar temporal trends, we used only LVG (MAN+GAL)−1 in the correlation analysis. LVG (MAN+GAL)−1 is negatively related to tundra-steppe taxa on a loglog scale (, p=0.07, Fig. 5) during MIS 8–5e, whereas LVG (MAN+GAL)−1 is negatively related to SGB (, p=0.07) during MIS 12–11c and has higher values not only during periods of high Sphagnum spore proportions (τ=0.7, p=0.047), especially during MIS 12–11c but also when considering all samples (Fig. 4b).
4.1 Preservation of monosaccharide anhydrides in high-latitude lake sediments
The abundance of LVG and its isomers in smoke in absolute (influxes) and relative (ratios) terms depends on the amount and type of biomass being burnt and on burning conditions (Engling et al., 2006; Kuo et al., 2008, 2011; Fabbri et al., 2009). Yet it is unknown, whether sedimentary MAs are suitable biomass burning proxies in Arctic lake sediments on centennial to orbital timescales. Issues such as analytical uncertainties, the source of MAs (i.e. geographic source area, type of biomass burnt and burning conditions) and degradation during transport, deposition and after deposition need to be addressed in order to use the proxies further back in time. We discuss the future potential of MA records in long-term high-latitude fire reconstructions and present some first ideas on past fire–vegetation–climate feedbacks on orbital and centennial to millennial timescales, as correlation was limited by the few samples that had both MA and > 0.5 % of certain pollen/NPP taxa. Although interglacial climate cannot be discussed independently here from vegetation composition, the main climate trends inferred from pollen-based vegetation reconstructions (Melles et al., 2012; Tarasov et al., 2013; Zhao et al., 2019) as described below have been supported by independent climate reconstructions based on sedimentary diatoms and biomarkers (Chapligin et al., 2012; D'Anjou et al., 2013).
4.1.1 Analytical uncertainties
MAs are present in up to 430 kyr old El'gygytgyn lake sediments and could be analysed well above the detection limit using U-HPLC high-resolution MS with average instrumental standard errors of 5 %–7 % (based on duplicate measurements). LVG influxes are of the same order of magnitude as found in other younger lake sediments from temperate and tropical regions (Schüpbach et al., 2015; Battistel et al., 2017; Callegaro et al., 2018; Dietze et al., 2019). This suggests that influx calculations based on the age–depth models of lake sediment cores PG1351 and 5011-1 are reasonable, despite absolute age uncertainties that are several hundreds of years (Nowaczyk et al., 2007, 2013).
We find a trend towards lower average MA influxes in older sediments (Fig. 2b), which is not as prominent when comparing the trajectories of MA influxes in time (Fig. 3a). This could indicate either a true signal of past fire activity, a certain degree of post-depositional degradation or a combination of both aspects. As there is no long-term trend visible in MA ratios (Fig. 2c), the positive relationship between MA isomers (Fig. 4a) suggests that they either derive from the same source and/or have been degraded in a similar way during transport and after deposition.
4.1.2 Potential source areas and degradation pathways from source to sink
While some formation and transport mechanisms are still uncertain (Suciu et al., 2019), MAs are thought be produced as gases during pyrolysis under low burning temperatures but quickly condensate on co-emitted particulate matter in the smoke plume. A small fraction of MAs can remain at the site and potentially adsorb to char during higher burning temperatures (ca. 600 ∘C), becoming available for subsequent transport by overland flows (Suciu et al., 2019). MAs oxidize via several degradation pathways during atmospheric transport and/or get lost by wet or dry deposition within hours to few days (see Suciu et al., 2019 for a review). Hence, we expect higher influxes when fires happen close to the lake. In central European lake sediments, large fire episodes ca. 20–100 km away from the deposit could be traced by robust MA peaks (Dietze et al., 2019). Here, we discuss the potential local and regional to extra-regional MA source areas, transport pathways and post-depositional degradation in El'gygytgyn lake sediments.
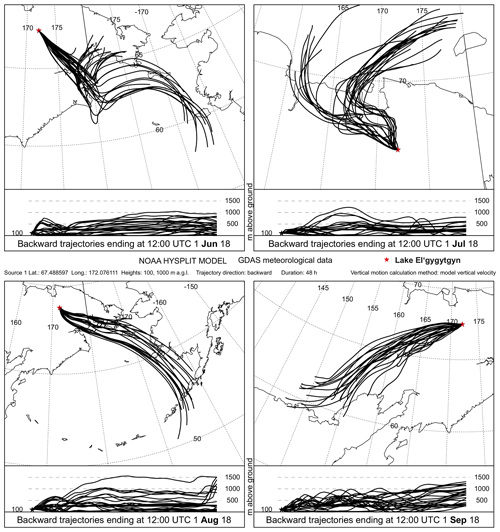
Figure 6Aerosol backward trajectories of summer 2018 as examples of potential modern analogues for source areas of burning residues being deposited in Lake El'gygytgyn (star) during interglacial summers. The HYSPLIT transport and dispersion model was kindly provided by the NOAA Air Resources Laboratory and the READY website (http://www.ready.noaa.gov, last access: 18 June 2019).
Local sources would require biomass burning within the lake catchment, which is rather small (183 km2) compared to the 110 km2 large lake (Nolan and Brigham-Grette, 2007). In the period 2000 to 2018, no fires have occurred in the El'gygytgyn catchment, and only a few occurred in the estimated pollen source area covering several hundreds of kilometres (based on remote sensing data, after Nitze et al., 2018). The sparse tundra vegetation is currently limited to slopes below 5∘ (Nolan and Brigham-Grette, 2007), which is ca. 55 % of the catchment. Hence, we suggest that during interglacials of similar or cooler conditions (e.g. during MIS 7e) catchment fires were highly unlikely. During warmer interglacials with a potential spread of boreal tree taxa towards the lake (Melles et al., 2012), we cannot exclude a certain contribution from local fires, but given the size of the lake we assume that the majority of MAs in El'gygytgyn sediments derive from extra-local aeolian transport.
Our HYSPLIT backward trajectories (Fig. 6) show that MAs attached to aerosols would derive from modern-day tundra and larch taiga in the Chukotka region, several hundreds of kilometres away from the lake, transported by north- to south-westerly winds during the main fire season in July and August, in agreement with modern climatology (Mock et al., 1998). Yet, El'gygytgyn MA influx records represent centennial-scale averages integrating over multiple fire events under multiple synoptic conditions that varied in the past, such as a changing jet stream position and orientation (Herzschuh et al., 2019). Hence, the potential source area could have been even larger during past interglacials but still be located in the vegetated realm of eastern Siberia. We assume that shifts in geographic source areas associated with shifts in atmospheric circulation would affect the variability in MA influxes within rather than between interglacials.
Beyond dry deposition, rain and snowfall can deposit MAs directly on the (frozen or unfrozen) lake surface and/or within the lake catchment. Snow and lake ice were found to be covered with partially black particulate matter (Melles et al., 2005), with snowmelt and ice-break up currently happening from mid-May to early July (Nolan et al., 2002), i.e. overlapping with the onset of the boreal fire season. Snowmelt causes high-energy fluvial transport across the alluvial fans that drain the catchment for few days and provide most of the detrital sediments to lake El'gygytgyn (Nolan and Brigham-Grette, 2007). The amount of local aeolian reworking of sediment from barren to sparsely vegetated surfaces during the snow-free season is unknown, but wind intensities are high throughout the year (Nolan and Brigham-Grette, 2007; Fedorov et al., 2013). Hence, a certain amount of MAs from within the catchment could have reached the lake in either dissolved or particulate form, e.g. adsorbed to clays (Suciu et al., 2019).
During interglacials, rather short residence times on snow and quick transport of MAs as part of the turbulent discharge of suspended matter in the main channels (Wennrich et al., 2013) could have (a) prevented an important contribution (and potential loss) of dissolved MAs to the local groundwater and (b) limited degradation during short distance fluvial transport (Hunsinger et al., 2008; Suciu et al., 2019). During warmer interglacials (MIS 5e and MIS 11c), a denser local vegetation coverage would have prolonged the snowmelt period and reduced the fluvial transport energies, also during rainstorms in summer. These effects would reduce the absolute fluvial and aeolian influxes of MAs from the catchment in warmer compared to cooler interglacials, which might be counterbalanced by an increased likelihood for more local fires during warmer interglacials. During glacials, climate and vegetation reconstructions suggest a dry and windy climate with a multiyear to perennially frozen lake surface and limited local runoff (Nolan et al., 2002; Melles et al., 2007, 2012), limiting also the influxes of MAs. Aeolian material, including extra-regional MAs, would experience much slower deposition times via moats and cracks as currently observed in Antarctica (Rivera-Hernandez et al., 2019), with enough time for certain not-well-constrained cryogenic processes to degrade MAs (Suciu et al., 2019).
MA degradation can also happen in the lake water column or after deposition. Recently, Schreuder et al. (2018) found that LVG was transported and settled attached to organic matter, which might have prevented its degradation within the marine water column, despite its water solubility. In contrast, Norwood et al. (2013) suggested a substantial MA degradation in well-oxygenated river water, which, however, could have been limited when MAs deposit quickly (Suciu et al., 2019). Yet, degradation and desorption of MAs at the sediment–water interface could still be substantial (Schreuder et al., 2018), especially under aerobic conditions (Knicker et al., 2013), as MAs are anhydrous sugars and, thus, potentially more labile and mobile than other organic compounds.
Monitoring and sediment properties of Lake El'gygytgyn suggest rapid depositional processes in a turbulent, wind-mixed water column (Wennrich et al., 2013) with well-oxygenated bottom waters during summers and past warm periods (Wennrich et al., 2013; Melles et al., 2012). During glacial periods long-term lake stratification led to anoxic bottom water conditions that improved the preservation of total organic carbon (Melles et al., 2007, 2012). Assuming that dissolved MAs degrade within days or weeks in oxic, turbulent water (Norwood et al., 2013), the MAs recorded from previous warm periods may rather derive from MAs in particulate phase, which probably did not experience post-depositional migration and which also cannot be produced by diagenesis (Suciu et al., 2019). If we assume a constant influx of particulate-phase MAs and high organic matter degradation at the lake bottom, we would expect higher preservation during glacials than interglacials – but we find higher MA influxes in interglacial sediments (Fig. 2b). Hence, we assume that MA degradation was limited over a longer time, when occluded within or adsorbed to a mineral matrix or iron oxides (Lalonde et al., 2012; Hemingway et al., 2019). MAs are known to adsorb well to minerals and organic particles such as co-emitted soot and are chemically prone to form organo-metal complexes via chelation (Tobo et al., 2012; Suciu et al., 2019). Adsorption (protecting) and desorption (destabilizing) processes can happen already during emission, transport and at the sediment–water interface, most likely in a rather short time of days to weeks (Suciu et al., 2019) and may vary with climate conditions, as described above. These short-term processes would affect all isomers in a similar way, and all isomers show indeed the same trends over centennial and orbital timescales (Fig. 2b), which is indicative for bound and protected compounds (Hemingway et al., 2019). Hence, we propose that MA influxes from El'gygytgyn sediments represent particle-bound MAs that are only marginally affected by degradation on centennial to orbital timescales, and, instead, represent relative changes in biomass burnt from a regional source during low-intensity fires.
4.2 Long-term relationships between low-intensity fires, climate and vegetation
4.2.1 Orbital-scale fire occurrence and the role of biomass and fuel availability, insolation and climate
More low-intensity biomass burning occurs during interglacials compared to glacials, as we find a tendency towards higher MA influxes during interglacials compared to the preceding late glacials. Considering source areas and transport pathways (Sect. 4.1.2), we suggest that during interglacials more biomass has burnt closer to Lake El'gygytgyn (Fig. 2b). Absolute biomass availability and land cover change cannot be assessed quantitatively from pollen percentage data due to (partly) unknown taxa-specific pollen and NPP dispersal, productivity and preservation (Sugita, 2007), and our low sample resolution prevents the use of new quantitative land cover reconstructions (Theuerkauf and Couwenberg, 2018). However, more biomass availability is suggested by significantly higher abundance of tree and shrub pollen in interglacial sediments compared to glacial cold tundra-steppe assemblages dominated by herbs and grasses (Fig. 5) (Melles et al., 2012; Tarasov et al., 2013). Accordingly, our rank correlation analysis shows that MA influxes tend to increase with higher amounts of tree and shrub versus tundra-steppe pollen (Figs. 3c, 4b) – potentially also allowing fires to occur in the El'gygytgyn catchment during warmer-than-present interglacials (MIS 5e and MIS 11c). During glacials, in contrast, low temperatures and CO2 levels limit biomass (fuel availability) and fire spread, which has be shown in previous mid- to high-latitude reconstructions and model simulations (Thonicke et al., 2005; Krawchuk and Moritz, 2011; Daniau et al., 2012; Martin Calvo et al., 2014; Kappenberg et al., 2019). Thus, glacial Lake El'gygytgyn MA influxes represent a background signal from remote source areas, when fires associated with high-productivity biomes have shifted southwards.
We did not find evidence for more biomass burning close to the lake in times of high summer insolation, as suggested by previous mid- to high-latitude studies (Daniau et al., 2012; Remy et al., 2017; Dietze et al., 2018; Kappenberg et al., 2019). Yet, MA influxes peaked during transitions from high to low summer insolation and during the MIS 8 insolation maximum, despite pollen data suggesting rather low tree and shrub presence during MIS 8 (Fig. 3a, c). In addition, lower mean MA influxes during MIS 11c compared to MIS 5e (Fig. 2b) might be linked to the rather moderate summer insolation during MIS 11 compared to a more pronounced insolation cycle during MIS 5e (Yin and Berger, 2012). Indirectly, high-latitude summer insolation could have driven biomass productivity and fires in the source areas, for example, not only by altering the length of the growing season but also by affecting land-atmosphere feedbacks and potential interhemispheric ice–ocean–land feedbacks that alter regional precipitation-evaporation patterns (Yin and Berger, 2012; Melles et al., 2012; Martin Calvo et al., 2014).
While it is difficult to fully disentangle biomass availability and climate conditions, we suggest that climate was the main driver of boreal forest fires on orbital timescales. This confirms previous studies from Holocene reconstructions, when, depending on regional biome configurations, wildfires have increased with increasing temperature and reduced precipitation patterns (Anderson et al., 2006; Remy et al., 2017; Molinari et al., 2018).
4.2.2 Centennial- to millennial-scale fire occurrence and the role of climate and vegetation composition
The differences in MA influxes among interglacials seem to reflect centennial- to millennial-scale temperature and moisture changes, as we find the lowest interglacial MA influxes during MIS 7e, known to be the coolest of the three interglacials considered here, when pollen of a birch and willow shrub tundra prevailed with little abundance of summergreen boreal and pine pollen (Lozhkin et al., 2007; Zhao et al., 2019) (Figs. 2b, 3a, 5). Yet, insolation and temperature control alone cannot explain the lower MA influxes during the super-interglacial MIS 11c compared to MIS 5e.
Regional moisture availability and biome configuration, hence fuel composition, seem to affect low-temperature fire occurrence on centennial to millennial scales for two reasons. First, we find a negative relationship between MA influxes and Sphagnum spores across all samples and especially during late MIS 12 and early MIS 11c (Fig. 4b), when Sphagnum spore abundance was highest (Fig. 5), indicating widespread peatlands. The presence of spruce pollen also confirmed that MIS 11c was wetter than MIS 5e (Melles et al., 2012), suggesting that fuel wetness limits fires on centennial timescales.
Second, most biomass was burnt (i.e. highest MA influxes) during the warm and dry MIS 5e, which was dominated by summergreen boreal (larch) and pine forest (Lozhkin et al., 2007; Melles et al., 2012). Our rank correlation analysis suggests a significant positive correlation of MA influxes with summergreen boreal tree pollen during MIS 8–5e (Fig. 4b). In addition, during the late MIS 12 and early MIS 11c, MA influxes peaked at the time of high summergreen boreal pollen, before spruce pollen reached their maximum and after the maximum in Sphagnum spore abundance (Fig. 3a, c). As there was also no relationship between spruce pollen and MA influxes (Fig. 4b), we suggest that evergreen spruce forest was not an important source of MAs on long timescales, in contrast to summergreen boreal forest.
There is also a tendency towards higher MA influxes with increasing pine pollen abundance during MIS 8–5e (Fig. 3a, c), whereas during MIS 12–11c Pinus s/g. Haploxylon-type pollen was not or weakly negatively related to MA influxes (τ not significant; Fig. 4b), maybe because different pine species with different fire-related traits could have produced the P. s/g. Haploxylon-type pollen during the two periods. The shrubby P. pumila that dominates in modern treeline ecotones does not survive frequent low-intensity surface fires, whereas P. sibirica, occurring in evergreen and high-elevation boreal forest, is not adapted to but able to survive fires (Ma et al., 2008; Keeley, 2012). Pinus s/g. Diploxylon-type pollen, derived from P. sylvestris, are almost absent in Lake El'gygytgyn sediments. However, as independent high-resolution climate proxy data are lacking for our samples, we cannot fully disentangle the role of vegetation composition and centennial- to millennial-scale climate conditions.
4.2.3 Centennial- to millennial-scale burning conditions: importance of the understorey
The relationships between MA influxes, summergreen and evergreen boreal taxa and Sphagnum are independently confirmed by MA ratios that depend on the type of biomass burnt and on burning conditions such as duration (Engling et al., 2006; Kuo et al., 2008, 2011; Fabbri et al., 2009). Before relating the MA influx-based with the MA ratio-based evidence, we have to state that, surprisingly, El'gygytgyn MAN and GAL influxes were up to 10 times higher than LVG influxes during all periods, and LVG MAN−1 and LVG (MAN+GAL)−1 ratios were much lower than 3 and 1, respectively (Fig. 2c). This is in contrast to previous observations and experimental burnings (Fig. 2d) that report MA ratios of about an order of magnitude higher than those found here (Oros and Simoneit, 2001a, b; Oros et al., 2006; Iinuma et al., 2007; Fabbri et al., 2009). El'gygytgyn MA ratios are also about 2 to 10 times lower than those previously reported for other lake systems (Kirchgeorg et al., 2014; Schüpbach et al., 2015; Callegaro et al., 2018; Dietze et al., 2019), suggesting that long-term post-depositional degradation could have altered MA ratios. However, the direction of diagenetic decomposition was opposite to what we find, i.e. no MAN and GAL in Miocene and older deposits (Fabbri et al., 2009; Marynowski et al., 2018; Suciu et al., 2019) As biomass differs in its relative proportions of cellulose (LVG precursor), hemicellulose (MAN and GAL precursor), lignin and others components (Simoneit et al., 1999; Simoneit, 2002), the emission rates of MA isomers differ, with a tendency towards highest MA ratios (i.e. high LVG production) in grasses, followed by deciduous hardwood trees, whereas coniferous softwood trees are characterized by relatively low MA ratios (Oros and Simoneit, 2001a, b; Engling et al., 2006; Oros et al., 2006; Schmidl et al., 2008; Jung et al., 2014).
Only Oros and Simoneit (2001b) and Otto et al. (2006) report similarly low MA ratios from burning of a mixed bark, needle, cone and wood sample of a temperate pine (Pinus monticola) and from a charred pine cone (P. banksiana), respectively. Yet, these softwood tree species are not present in Siberia, and there is no relation between MA ratios and pine pollen during MIS 8–5e but rather a tendency towards higher ratios LVG (MAN+GAL)−1 with increased pine during MIS 12–11c – which cannot explain the low El'gygytgyn MA ratios. There was also no relationship between MA ratios and spruce pollen in our samples, supporting the suggestion from MA influx-based evidence that evergreen conifers do not significantly influence El'gygytgyn MA records.
In the studied MIS 12–11c samples, Sphagnum is significantly positively related to LVG (MAN+GAL)−1, with highest ratios not only in times of highest Sphagnum abundance but also in times of very low background MA influxes (Figs. 3b, c; 4b). LVG MAN−1 ratios in modern-day aerosols of peatland fires in Russia were found to be > 7 (Fujii et al., 2014), that is, higher than softwood-derived MA ratios but lower than those from hardwoods and grasses (Fig. 2d) – which cannot explain the low El'gygytgyn MA ratios.
Instead, we find a significant negative correlation between El'gygytgyn MA ratios and the summergreen boreal pollen sum during MIS 12–11c, confirming reports that burning of larch wood produce relatively higher levels of GAL (= lower LVG (MAN+GAL)−1 ratios) than other softwoods (Schmidl et al., 2008). The reported larch LVG MAN−1 ratios, however, are still about 1.8 to 5 times higher than what we have measured. In addition, we find low ratios across all samples, mainly independent of high or low influxes. Hence, we hypothesize that an additional biomass source and/or specific burning conditions have contributed to the low sedimentary MA ratios found in Lake El'gygytgyn sediments.
One additional biomass source could be dense moss–lichen mats within the summergreen boreal forest, assuming that past ecosystem properties were similar as today. Light can penetrate more easily through the open canopy of summergreen compared to evergreen boreal forest, enabling the understorey to dry up quickly during summer droughts. The more open the canopy is, the denser the moss–lichen mats in the understorey become, providing several percent of the total biomass and carbon stored in NE Siberian larch forests and supporting the insulation the underlain permafrost (Isaev et al., 2010; Loranty et al., 2018). Together with larch needles and deadwood, moss–lichen layers are highly flammable, promoting fast surface fires, because winds can penetrate easily to the forest floor under an open canopy (Sofronov et al., 2004). Although we could not find any literature on MA emissions after burning of mosses (other than Sphagnum) and lichens, their cell walls are also composed of cellulose and hemicellulose (Honegger and Bartnicki-Garcia, 1991; Roberts et al., 2012) – suggesting them as a likely source of MAs. As cellulose and hemicellulose have slightly different thermal stabilities (Yang et al., 2007), different proportions in moss–lichen mats might have favoured the release of hemicellulose-derived MAN and GAL compared to LVG favoured in woody biomass burning.
Specific burning conditions can favour MAN and GAL over LVG release. In experiments, MAN and GAL reach higher yields at slightly lower temperatures (ca. 200 ∘C) compared to LVG (ca. 250 ∘C), with a strong decline in overall MA yields at temperatures higher than 350 ∘C (Engling et al., 2006; Kuo et al., 2008, 2011; Knicker et al., 2013). Although average burn temperatures of low-intensity surface fires in larch forests are unknown, the suggested high speed of surface fires under open canopies (Sofronov et al., 2004) would decrease burn durations, with absolute MA yields increasing during shorter burn durations (Engling et al., 2006; Kuo et al., 2011). After burning of the moss–lichen layer, rejuvenation of larch and tree growth is promoted by active–layer thickening and nutrient release for several decades post fire, until moss–lichen mats recover (Loranty et al., 2018).
Overall, all samples and sedimentary MA influxes and ratios reported here are integrated over centennial to millennial timescales. Together with the MA influx-based evidence (i.e. no significant differences and very low MA ratios across glacial-interglacial periods, Fig. 2c), we propose that during all times there was a high contribution of MAs from low-temperature surface fires in summergreen larch forest presumably including the burning of moss–lichen mats of currently unknown MA emissions ratios. In periods of low MA influxes (background influxes), higher MA ratios probably included more burning residues from remote grass, peatland and forest fires, whereas higher influxes suggest that summergreen boreal fires happened closer to Lake El'gygytgyn – with the northward spread of larch forest being well-documented in MIS 11c and 5e pollen records (Lozhkin et al., 2007, 2017; Melles et al., 2012).
Considering a future warming and wetting of the high northern latitudes (Hoegh-Guldberg et al., 2018), we would expect an increase in the availability of flammable biomass on long timescales, but low-intensity fire might decrease when fuel moisture exceeds a certain threshold – by either transition from a stable forest state to peatlands or shifts from summergreen to evergreen boreal forest – despite potentially increasing fire ignitions (Veraverbeke et al., 2017).
Molecular proxies are increasingly being used in palaeoenvironmental studies, providing insights into past biogeochemical cycles during periods that provide natural analogues of the expected future regional change. Here, we have shown the potential of MA influxes and ratios in high northern lake sediments as proxies for the amount and type of low-intensity biomass burning. Although limited in samples, we can deduce first fire–climate–vegetation relationships in north-eastern Siberia on long timescales, which can provide guidance towards a natural, process-based land management of the future.
MAs can be measured well above the detection limit using U-HPLC-HRMS on sediment samples of Lake El'gygytgyn for three previous glacial-to-interglacial periods and seem little affected by degradation. A declining trend in MA influxes with time is thought to represent the past amount of biomass burnt during low-temperature fires that can be related to climate, regional biomass availability and biomass composition on orbital and centennial to millennial timescales.
Low-temperature fires are an important component of the fire regime and biogeochemical cycles of modern Siberian larch forests (Kharuk et al., 2011; Rogers et al., 2015; Chen and Loboda, 2018), a relationship that seems to hold on centennial to millennial timescales during past interglacials.
Relatively higher MA influxes during interglacials and times of high summer insolation suggest that low-temperature fires are closely linked to biomass availability and climate conditions that favour fuel dryness on orbital timescales. Differences between interglacials are revealed by higher MA influxes when summergreen boreal forest has spread closer towards Lake El'gygytgyn, although there is no clear relationship with evergreen coniferous taxa.
Surprisingly high influxes of MAN and GAL compared to LVG (i.e. low MA ratios across all periods) cannot be explained solely by woody biomass burning. We hypothesize that MA can serve as a proxy for fuels that derive primarily from understorey and moss–lichen mats, typical of open-canopy larch forests, that have shifted their geographic distribution southwards during glacial times.
Further research will continue exploring lake-sedimentary MAs in higher resolution together with further sedimentary fire proxies such as charcoal to study low-intensity fire–climate–vegetation feedbacks in space and time and potential ways of post-depositional degradation in even older interglacials, with CO2 levels similar to those expected in the future.
Data are available at https://doi.org/10.1594/PANGAEA.915603 (Dietze et al., 2020) and https://doi.org/10.1594/PANGAEA.915749 (Andreev et al., 2020).
The study was conceptualized by ED, UH and KM. Method development and lab analysis were performed by ED, CK and AA, with support by ECH, LTS, OR and DS. ED analysed the data, with further data support by VW. ED wrote the paper with contributions from all authors.
The authors declare that they have no conflict of interest.
We dedicate this study to Sophie Stehle†, who assisted in the lab and left us too early. We thank also Denise Dorhout for lab assistance, Norbert Nowaczyk, Stefanie Poetz, Niels Hovius, Georg Schwamborn and Stefan Kruse for discussions, and Cathy Jenks for English editing.
This research has been supported by the DFG German Research Foundation (grant no. DI 2544/1-1, 419058007). Andrei Andreev was partly funded by the
Russian Government Program of Competitive Growth of Kazan Federal
University.
The article processing charges for this open-access
publication were covered by a Research
Centre of the Helmholtz Association.
This paper was edited by Zhengtang Guo and reviewed by John Dodson and one anonymous referee.
Abbott, B., W., Jones, J., B., Schuur, E., A. G., Chapin, F. S., Bowden, W. B., Bret-Harte, M. S., Epstein, H. E., Flannigan, M., D., Harms, T. K., Hollingsworth, T. N., Mack, M. C., McGuire, A. D., Natali, S. M., Rocha, A. V., Tank, S. E., Turetsky, M. R., Vonk, J. E., Wickland, K. P., Aiken, G. R., Alexander, H. D., Amon, R. M. W., Benscoter, B. W., Bergeron, Y., Bishop, K., Blarquez, O., Bond-Lamberty, B., Breen, A. L., Buffam, I., Cai, Y., Carcaillet, C., Carey, S. K., Jing, M. C., Han, Y. H. C., Christensen, T. R., Cooper, L. W., Cornelissen, J. H. C., de Groot, W. J., DeLuca, T. H., Dorrepaal, E., Ned, F., Jacques, C. F., Bruce, C. F., Nancy, H. F. F., Sylvie, G., Martin, P. G., Scott, J. G., Johann, G. G., Laura, G., Paul, G., Laodong, G., Higuera, P. E., Larry, H., Feng Sheng, H., Gustaf, H., Elchin, E. J., Randi, J., Jill, F. J., Jan, K., Eric, S. K., Gerhard, K., Ryan, K., Frida, K., George, W. K., Pirkko, K., Jari, K., Peter, K., Hjalmar, L., Isabelle, L., Robie, W. M., Paul, J. M., Pertti, J. M., James, W. M., Ulf, M., Steven, F. O., David, O., David, P., Marc-André, P., Payette, S., Peng, C., Pokrovsky, O. S., Rastetter, E. B., Raymond, P. A., Raynolds, M. K., Rein, G., Reynolds, J. F., Robards, M., Rogers, B. M., Schädel, C., Schaefer, K., Schmidt, I. K., Shvidenko, A., Sky, J., Spencer, R. G. M., Starr, G., Striegl, R. G., Teisserenc, R., Tranvik, L. J., Virtanen, T., Welker, J. M., and Zimov, S.: Biomass offsets little or none of permafrost carbon release from soils, streams, and wildfire: an expert assessment, Environ. Res. Lett., 11, 034014, https://doi.org/10.1088/1748-9326/11/3/034014, 2016.
Aitchison, J.: The statistical analysis of compositional data, Chapham and Hall, London, New York, 1986.
Anderson, R. S., Hallett, D. J., Berg, E., Jass, R. B., Toney, J. L., de Fontaine, C. S., and DeVolder, A.: Holocene development of Boreal forests and fire regimes on the Kenai Lowlands of Alaska, Holocene, 16, 791–803, https://doi.org/10.1191/0959683606hol966rp, 2006.
Andreev, A. A., Morozova, E., Fedorov, G., Schirrmeister, L., Bobrov, A. A., Kienast, F., and Schwamborn, G.: Vegetation history of central Chukotka deduced from permafrost paleoenvironmental records of the El'gygytgyn Impact Crater, Clim. Past, 8, 1287–1300, https://doi.org/10.5194/cp-8-1287-2012, 2012.
Andreev, A. A., Tarasov, P. E., Wennrich, V., Raschke, E., Herzschuh, U., Nowaczyk, N. R., Brigham-Grette, J., and Melles, M.: Late Pliocene and Early Pleistocene vegetation history of northeastern Russian Arctic inferred from the Lake El'gygytgyn pollen record, Clim. Past, 10, 1017–1039, https://doi.org/10.5194/cp-10-1017-2014, 2014.
Andreev, A. A., Tarasov, P. E., Wennrich, V., and Melles, M.: Millennial-scale vegetation changes in the north-eastern Russian Arctic during the Pliocene/Pleistocene transition (2.7–2.5 Ma) inferred from the pollen record of Lake El'gygytgyn, Quaternary Sci. Rev., 147, 245–258, https://doi.org/10.1016/j.quascirev.2016.03.030, 2016.
Andreev, A. A., Dietze, E., and Herzschuh, U.: Pollen data of Lake El'gygytgyn sediments, core PG1351 (MIS 5e, 6, 7e, 8), PANGAEA, https://doi.org/10.1594/PANGAEA.915749, 2020.
Archibald, S., Lehmann, C. E. R., Gómez-Dans, J. L., and Bradstock, R. A.: Defining pyromes and global syndromes of fire regimes, P. Natl. Acad. Sci. USA, 110, 6442–6447, https://doi.org/10.1073/pnas.1211466110, 2013.
Arndt, S., Turvey, C., and Andreasen, N. C.: Correlating and predicting psychiatric symptom ratings: Spearmans r versus Kendalls tau correlation, J. Psychiat. Res., 33, 97–104, https://doi.org/10.1016/S0022-3956(98)90046-2, 1999.
Battistel, D., Argiriadis, E., Kehrwald, N., Spigariol, M., Russell, J. M., and Barbante, C.: Fire and human record at Lake Victoria, East Africa, during the Early Iron Age: Did humans or climate cause massive ecosystem changes?, Holocene, 27, 997–1007, https://doi.org/10.1177/0959683616678466, 2017.
Bauer, D. F.: Constructing Confidence Sets Using Rank Statistics, J. Am. Stat. Assoc., 67, 687–690, https://doi.org/10.1080/01621459.1972.10481279, 1972.
Brigham-Grette, J., Melles, M., Minyuk, P., Andreev, A., Tarasov, P., DeConto, R., Koenig, S., Nowaczyk, N., Wennrich, V., Rosén, P., Haltia, E., Cook, T., Gebhardt, C., Meyer-Jacob, C., Snyder, J., and Herzschuh, U.: Pliocene Warmth, Polar Amplification, and Stepped Pleistocene Cooling Recorded in NE Arctic Russia, Science, 340, 1421–1427, https://doi.org/10.1126/science.1233137, 2013.
Buchholz, G., Goldammer, J. G., and Martell, D. L.: Application of Logistic Models to Predict Human-Caused Forest Fires in Siberia, in: Early Warning Systems for Natural Disaster Reduction, edited by: Zschau, J. and Küppers, A., Springer Berlin Heidelberg, Berlin, Heidelberg, 593–599, 2003.
Callegaro, A., Battistel, D., Kehrwald, N. M., Matsubara Pereira, F., Kirchgeorg, T., Villoslada Hidalgo, M. D. C., Bird, B. W., and Barbante, C.: Fire, vegetation, and Holocene climate in a southeastern Tibetan lake: a multi-biomarker reconstruction from Paru Co, Clim. Past, 14, 1543–1563, https://doi.org/10.5194/cp-14-1543-2018, 2018.
Chapligin, B., Meyer, H., Swann, G. E. A., Meyer-Jacob, C., and Hubberten, H.-W.: A 250 ka oxygen isotope record from diatoms at Lake El'gygytgyn, far east Russian Arctic, Clim. Past, 8, 1621–1636, https://doi.org/10.5194/cp-8-1621-2012, 2012.
Chen, D. and Loboda, T. V.: Surface forcing of non-stand-replacing fires in Siberian larch forests, Environ. Res. Lett., 13, 045008, https://doi.org/10.1088/1748-9326/aab443, 2018.
Conedera, M., Tinner, W., Neff, C., Meurer, M., Dickens, A. F., and Krebs, P.: Reconstructing past fire regimes: methods, applications, and relevance to fire management and conservation, Quaternary Sci. Rev., 28, 555–576, https://doi.org/10.1016/j.quascirev.2008.11.005, 2009.
Daniau, A. L., Bartlein, P. J., Harrison, S. P., Prentice, I. C., Brewer, S., Friedlingstein, P., Harrison-Prentice, T. I., Inoue, J., Izumi, K., Marlon, J. R., Mooney, S., Power, M. J., Stevenson, J., Tinner, W., Andrič, M., Atanassova, J., Behling, H., Black, M., Blarquez, O., Brown, K. J., Carcaillet, C., Colhoun, E. A., Colombaroli, D., Davis, B. A. S., D'Costa, D., Dodson, J., Dupont, L., Eshetu, Z., Gavin, D. G., Genries, A., Haberle, S., Hallett, D. J., Hope, G., Horn, S. P., Kassa, T. G., Katamura, F., Kennedy, L. M., Kershaw, P., Krivonogov, S., Long, C., Magri, D., Marinova, E., McKenzie, G. M., Moreno, P. I., Moss, P., Neumann, F. H., Norström, E., Paitre, C., Rius, D., Roberts, N., Robinson, G. S., Sasaki, N., Scott, L., Takahara, H., Terwilliger, V., Thevenon, F., Turner, R., Valsecchi, V. G., Vannière, B., Walsh, M., Williams, N., and Zhang, Y.: Predictability of biomass burning in response to climate changes, Global Biogeochem. Cy., 26, GB4007, https://doi.org/10.1029/2011GB004249, 2012.
D'Anjou, R. M., Wei, J. H., Castañeda, I. S., Brigham-Grette, J., Petsch, S. T., and Finkelstein, D. B.: High-latitude environmental change during MIS 9 and 11: biogeochemical evidence from Lake El'gygytgyn, Far East Russia, Clim. Past, 9, 567–581, https://doi.org/10.5194/cp-9-567-2013, 2013.
Dietze, E., Theuerkauf, M., Bloom, K., Brauer, A., Dörfler, W., Feeser, I., Feurdean, A., Gedminienė, L., Giesecke, T., Jahns, S., Karpińska-Kołaczek, M., Kołaczek, P., Lamentowicz, M., Latałowa, M., Marcisz, K., Obremska, M., Pędziszewska, A., Poska, A., Rehfeld, K., Stančikaitė, M., Stivrins, N., Święta-Musznicka, J., Szal, M., Vassiljev, J., Veski, S., Wacnik, A., Weisbrodt, D., Wiethold, J., Vannière, B., and Słowiński, M.: Holocene fire activity during low-natural flammability periods reveals scale-dependent cultural human-fire relationships in Europe, Quaternary Sci. Rev., 201, 44–56, https://doi.org/10.1016/j.quascirev.2018.10.005, 2018.
Dietze, E., Brykała, D., Schreuder, L. T., Jażdżewski, K., Blarquez, O., Brauer, A., Dietze, M., Obremska, M., Ott, F., Pieńczewska, A., Schouten, S., Hopmans, E. C., and Słowiński, M.: Human-induced fire regime shifts during 19th century industrialization: A robust fire regime reconstruction using northern Polish lake sediments, PLOS ONE, 14, e0222011, https://doi.org/10.1371/journal.pone.0222011, 2019.
Dietze, E., Karger, C., and Mangelsdorf, K.: Monosaccharide anhydrides (MA) records of Lake El'gygytgyn sediments (MIS 5e, 6, 7e, 8,11c, 12c), PANGAEA, https://doi.org/10.1594/PANGAEA.915603, 2020.
Elias, V. O., Simoneit, B. R. T., Cordeiro, R. C., and Turcq, B.: Evaluating levoglucosan as an indicator of biomass burning in Carajás, amazônia: a comparison to the charcoal record2, Geochim. Cosmochim. Ac., 65, 267–272, https://doi.org/10.1016/S0016-7037(00)00522-6, 2001.
Engling, G., Carrico, C. M., Kreidenweis, S. M., Collett Jr, J. L., Day, D. E., Malm, W. C., Lincoln, E., Min Hao, W., Iinuma, Y., and Herrmann, H.: Determination of levoglucosan in biomass combustion aerosol by high-performance anion-exchange chromatography with pulsed amperometric detection, Atmos. Environ., 40, 299–311, https://doi.org/10.1016/j.atmosenv.2005.12.069, 2006.
Fabbri, D., Torri, C., Simoneit, B. R. T., Marynowski, L., Rushdi, A. I., and Fabiańska, M. J.: Levoglucosan and other cellulose and lignin markers in emissions from burning of Miocene lignites, Atmos. Environ., 43, 2286–2295, https://doi.org/10.1016/j.atmosenv.2009.01.030, 2009.
Fedorov, G., Nolan, M., Brigham-Grette, J., Bolshiyanov, D., Schwamborn, G., and Juschus, O.: Preliminary estimation of Lake El'gygytgyn water balance and sediment income, Clim. Past, 9, 1455–1465, https://doi.org/10.5194/cp-9-1455-2013, 2013.
Feurdean, A., Vannière, B., Finsinger, W., Warren, D., Connor, S. C., Forrest, M., Liakka, J., Panait, A., Werner, C., Andrič, M., Bobek, P., Carter, V. A., Davis, B., Diaconu, A.-C., Dietze, E., Feeser, I., Florescu, G., Gałka, M., Giesecke, T., Jahns, S., Jamrichová, E., Kajukało, K., Kaplan, J., Karpińska-Kołaczek, M., Kołaczek, P., Kuneš, P., Kupriyanov, D., Lamentowicz, M., Lemmen, C., Magyari, E. K., Marcisz, K., Marinova, E., Niamir, A., Novenko, E., Obremska, M., Pędziszewska, A., Pfeiffer, M., Poska, A., Rösch, M., Słowiński, M., Stančikaitė, M., Szal, M., Święta-Musznicka, J., Tanţău, I., Theuerkauf, M., Tonkov, S., Valkó, O., Vassiljev, J., Veski, S., Vincze, I., Wacnik, A., Wiethold, J., and Hickler, T.: Fire hazard modulation by long-term dynamics in land cover and dominant forest type in eastern and central Europe, Biogeosciences, 17, 1213–1230, https://doi.org/10.5194/bg-17-1213-2020, 2020.
Fujii, Y., Iriana, W., Oda, M., Puriwigati, A., Tohno, S., Lestari, P., Mizohata, A., and Huboyo, H. S.: Characteristics of carbonaceous aerosols emitted from peatland fire in Riau, Sumatra, Indonesia, Atmos. Environ., 87, 164–169, https://doi.org/10.1016/j.atmosenv.2014.01.037, 2014.
Furyaev, V. V., Vaganov, E. A., Tchebakova, N. M., and Valendik, E. N.: Effects of Fire and Climate on Successions and Structural Changes in The Siberian Boreal Forest, Eurasian Journal of Forest Research, 2, 1–15, 2001.
Gao, S., Hegg, D. A., Hobbs, P. V., Kirchstetter, T. W., Magi, B. I., and Sadilek, M.: Water-soluble organic components in aerosols associated with savanna fires in southern Africa: Identification, evolution, and distribution, J. Geophys. Res.-Atmos., 108, 8491, https://doi.org/10.1029/2002jd002324, 2003.
Gonzalez, P., Neilson, R. P., Lenihan, J. M., and Drapek, R. J.: Global patterns in the vulnerability of ecosystems to vegetation shifts due to climate change, Global Ecol. Biogeogr., 19, 755–768, https://doi.org/10.1111/j.1466-8238.2010.00558.x, 2010.
Han, Y. M., Peteet, D. M., Arimoto, R., Cao, J. J., An, Z. S., Sritrairat, S., and Yan, B. Z.: Climate and Fuel Controls on North American Paleofires: Smoldering to Flaming in the Late-glacial-Holocene Transition, Sci. Rep.-UK, 6, 20719, https://doi.org/10.1038/srep20719, 2016.
Harris, R. M. B., Remenyi, T. A., Williamson, G. J., Bindoff, N. L., and Bowman, D. M. J. S.: Climate–vegetation–fire interactions and feedbacks: trivial detail or major barrier to projecting the future of the Earth system?, Wiley Interdisciplinary Reviews: Climate Change, 7, 910–931, https://doi.org/10.1002/wcc.428, 2016.
Hemingway, J. D., Rothman, D. H., Grant, K. E., Rosengard, S. Z., Eglinton, T. I., Derry, L. A., and Galy, V. V.: Mineral protection regulates long-term global preservation of natural organic carbon, Nature, 570, 228–231, https://doi.org/10.1038/s41586-019-1280-6, 2019.
Herzschuh, U., Birks, H. J. B., Laepple, T., Andreev, A., Melles, M., and Brigham-Grette, J.: Glacial legacies on interglacial vegetation at the Pliocene-Pleistocene transition in NE Asia, Nat. Commun., 7, 11967, https://doi.org/10.1038/ncomms11967, 2016.
Herzschuh, U., Cao, X., Laepple, T., Dallmeyer, A., Telford, R. J., Ni, J., Chen, F., Kong, Z., Liu, G., Liu, K.-B., Liu, X., Stebich, M., Tang, L., Tian, F., Wang, Y., Wischnewski, J., Xu, Q., Yan, S., Yang, Z., Yu, G., Zhang, Y., Zhao, Y., and Zheng, Z.: Position and orientation of the westerly jet determined Holocene rainfall patterns in China, Nat. Commun., 10, 2376, https://doi.org/10.1038/s41467-019-09866-8, 2019.
Hoegh-Guldberg, O., Jacob, D., Taylor, M., Bindi, M., Brown, S., Camilloni, I., Diedhiou, A., Djalante, R., Ebi, K. L., Engelbrecht, F., Guiot, J., Hijioka, Y., Mehrotra, S., Payne, A., Seneviratne, S. I., Thomas, A., Warren, R., and Zhou, G.: Impacts of 1.5ºC Global Warming on Natural and Human Systems, in: Global Warming of 1.5°C. An IPCC Special Report on the impacts of global warming of 1.5°C above pre-industrial levels and related global greenhouse gas emission pathways, in the context of strengthening the global response to the threat of climate change, sustainable development, and efforts to eradicate poverty, edited by: Masson-Delmotte, V., Zhai, P., Pörtner, H.-O., Roberts, D., Skea, J., Shukla, P. R., Pirani, A., Moufouma-Okia, W., Péan, C., Pidcock, R., Connors, S., Matthews, J. B. R., Chen, Y., Zhou, X., Gomis, M. I., Lonnoy, E., Maycock, T., Tignor, M., and Waterfield, T., in Press, 2018.
Honegger, R. and Bartnicki-Garcia, S.: Cell wall structure and composition of cultured mycobionts from the lichens Cladonia macrophylla, Cladonia caespiticia, and Physcia stellaris (Lecanorales, Ascomycetes), Mycol. Res., 95, 905–914, https://doi.org/10.1016/S0953-7562(09)80085-3, 1991.
Hopmans, E. C., dos Santos, R. A. L., Mets, A., Sinninghe Damsté, J. S., and Schouten, S.: A novel method for the rapid analysis of levoglucosan in soils and sediments, Org. Geochem., 58, 86–88, https://doi.org/10.1016/j.orggeochem.2013.02.003, 2013.
Hu, F. S., Higuera, P. E., Walsh, J. E., Chapman, W. L., Duffy, P. A., Brubaker, L. B., and Chipman, M. L.: Tundra burning in Alaska: Linkages to climatic change and sea ice retreat, J. Geophys. Res.-Biogeo., 115, G04002, https://doi.org/10.1029/2009JG001270, 2010.
Hunsinger, G. B., Mitra, S., Warrick, J. A., and Alexander, C. R.: Oceanic loading of wildfire-derived organic compounds from a small mountainous river, J. Geophys. Res.-Biogeo., 113, G02007, https://doi.org/10.1029/2007jg000476, 2008.
Iinuma, Y., Brüggemann, E., Gnauk, T., Müller, K., Andreae, M. O., Helas, G., Parmar, R., and Herrmann, H.: Source characterization of biomass burning particles: The combustion of selected European conifers, African hardwood, savanna grass, and German and Indonesian peat, J. Geophys. Res.-Atmos., 112, J. Geophys. Res.-Biogeo., https://doi.org/10.1029/2006jd007120, 2007.
IPCC: Climate Change 2014: Impacts, Adaptation, and Vulnerability. Part B: Regional Aspects, in: Contribution of Working Group II to the Fifth Assessment Report of the Intergovernmental Panel on Climate Change, edited by: Barros, V. R., Field, C. B., Dokken, D. J., Mastrandrea, M. D., Mach, K. J., Bilir, T. E., Chatterjee, M., Ebi, K. L., Estrada, Y. O., Genova, R. C., Girma, B., Kissel, E. S., Levy, A. N., MacCracken, S., Mastrandrea, P. R., and White, L. L., Cambridge University Press, Cambridge, United Kingdom and New York, NY, USA, 688 pp., 2014.
Isaev, A. P., Protopopov, A. V., Protopopova, V. V., Egorova, A. A., Timofeyev, P. A., Nikolaev, A. N., Shurduk, I. F., Lytkina, L. P., Ermakov, N. B., Nikitina, N. V., Efimova, A. P., Zakharova, V. I., Cherosov, M. M., Nikolin, E. G., Sosina, N. K., Troeva, E. I., Gogoleva, P. A., Kuznetsova, L. V., Pestryakov, B. N., Mironova, S. I., and Sleptsova, N. P.: Vegetation of Yakutia: Elements of Ecology and Plant Sociology, in: The Far North: Plant Biodiversity and Ecology of Yakutia, edited by: Troeva, E. I., Isaev, A. P., Cherosov, M. M., and Karpov, N. S., Springer Netherlands, Dordrecht, 143–260, 2010.
Jackson, D. A. and Somers, K. M.: The spectre of “spurious” correlations, Oecologia, 86, 147–151, 1991.
Jung, J., Lee, S., Kim, H., Kim, D., Lee, H., and Oh, S.: Quantitative determination of the biomass-burning contribution to atmospheric carbonaceous aerosols in Daejeon, Korea, during the rice-harvest period, Atmos. Environ., 89, 642–650, https://doi.org/10.1016/j.atmosenv.2014.03.010, 2014.
Kappenberg, A., Lehndorff, E., Pickarski, N., Litt, T., and Amelung, W.: Solar controls of fire events during the past 600,000 years, Quaternary Sci. Rev., 208, 97–104, https://doi.org/10.1016/j.quascirev.2019.02.008, 2019.
Keeley, J. E.: Fire intensity, fire severity and burn severity: a brief review and suggested usage, Int. J. Wildland Fire, 18, 116–126, https://doi.org/10.1071/WF07049, 2009.
Keeley, J. E.: Ecology and evolution of pine life histories, Ann. For. Sci., 69, 445–453, https://doi.org/10.1007/s13595-012-0201-8, 2012.
Kharuk, V. I., Ranson, K. J., Dvinskaya, M. L., and Im, S. T.: Wildfires in northern Siberian larch dominated communities, Environ. Res. Lett., 6, 045208, https://doi.org/10.1088/1748-9326/6/4/045208, 2011.
Kirchgeorg, T., Schüpbach, S., Kehrwald, N., McWethy, D. B., and Barbante, C.: Method for the determination of specific molecular markers of biomass burning in lake sediments, Org. Geochem., 71, 1–6, https://doi.org/10.1016/j.orggeochem.2014.02.014, 2014.
Knicker, H., Hilscher, A., de la Rosa, J. M., González-Pérez, J. A., and González-Vila, F. J.: Modification of biomarkers in pyrogenic organic matter during the initial phase of charcoal biodegradation in soils, Geoderma, 197–198, 43–50, https://doi.org/10.1016/j.geoderma.2012.12.021, 2013.
Krawchuk, M. A. and Moritz, M. A.: Constraints on global fire activity vary across a resource gradient, Ecology, 92, 121–132, https://doi.org/10.1890/09-1843.1, 2011.
Kuo, L.-J., Herbert, B. E., and Louchouarn, P.: Can levoglucosan be used to characterize and quantify char/charcoal black carbon in environmental media?, Org. Geochem., 39, 1466–1478, https://doi.org/10.1016/j.orggeochem.2008.04.026, 2008.
Kuo, L.-J., Louchouarn, P., and Herbert, B. E.: Influence of combustion conditions on yields of solvent-extractable anhydrosugars and lignin phenols in chars: Implications for characterizations of biomass combustion residues, Chemosphere, 85, 797–805, https://doi.org/10.1016/j.chemosphere.2011.06.074, 2011.
Lalonde, K., Mucci, A., Ouellet, A., and Gélinas, Y.: Preservation of organic matter in sediments promoted by iron, Nature, 483, 198–200, https://doi.org/10.1038/nature10855, 2012.
Laskar, J., Robutel, P., Joutel, F., Gastineau, M., Correia, A. C. M., and Levrard, B.: A long-term numerical solution for the insolation quantities of the Earth, A&A, 428, 261–285, 2004.
Lasslop, G., Brovkin, V., Reick, C. H., Bathiany, S., and Kloster, S.: Multiple stable states of tree cover in a global land surface model due to a fire-vegetation feedback, Geophys. Res. Lett., 43, 6324–6331, https://doi.org/10.1002/2016gl069365, 2016.
Linderholm, H. W., Nicolle, M., Francus, P., Gajewski, K., Helama, S., Korhola, A., Solomina, O., Yu, Z., Zhang, P., D'Andrea, W. J., Debret, M., Divine, D. V., Gunnarson, B. E., Loader, N. J., Massei, N., Seftigen, K., Thomas, E. K., Werner, J., Andersson, S., Berntsson, A., Luoto, T. P., Nevalainen, L., Saarni, S., and Väliranta, M.: Arctic hydroclimate variability during the last 2000 years: current understanding and research challenges, Clim. Past, 14, 473–514, https://doi.org/10.5194/cp-14-473-2018, 2018.
Lisiecki, L. E. and Raymo, M. E.: A Pliocene-Pleistocene stack of 57 globally distributed benthic δ18O records, Paleoceanography, 20, A1003, https://doi.org/10.1029/2004PA001071, 2005.
Lopes dos Santos, R. A., De Deckker, P., Hopmans, E. C., Magee, J. W., Mets, A., Sinninghe Damste, J. S., and Schouten, S.: Abrupt vegetation change after the Late Quaternary megafaunal extinction in southeastern Australia, Nat. Geosci., 6, 627–631, https://doi.org/10.1038/ngeo1856, 2013.
Loranty, M. M., Natali, S. M., Berner, L. T., Goetz, S. J., Holmes, R. M., Davydov, S. P., Zimov, N. S., and Zimov, S. A.: Siberian tundra ecosystem vegetation and carbon stocks four decades after wildfire, J. Geophys. Res.-Biogeo., 119, 2144–2154, https://doi.org/10.1002/2014JG002730, 2014.
Loranty, M. M., Berner, L. T., Taber, E. D., Kropp, H., Natali, S. M., Alexander, H. D., Davydov, S. P., and Zimov, N. S.: Understory vegetation mediates permafrost active layer dynamics and carbon dioxide fluxes in open-canopy larch forests of northeastern Siberia, PLOS ONE, 13, e0194014, https://doi.org/10.1371/journal.pone.0194014, 2018.
Lozhkin, A. V., Anderson, P. M., Matrosova, T. V., and Minyuk, P. S.: The pollen record from El'gygytgyn Lake: implications for vegetation and climate histories of northern Chukotka since the late middle Pleistocene, J. Paleolimnol., 37, 135–153, https://doi.org/10.1007/s10933-006-9018-5, 2007.
Lozhkin, A. V., Minyuk, P. S., Anderson, P. M., Nedorubova, E. Y., and Korzun, J. V.: Variability in landscape and lake system responses to glacial and interglacial climates during the Middle Pleistocene based on palynological and geochemical data from Lake El'gygytgyn, Eastern Arctic, Rev. Palaeobot. Palyno., 246, 1–13, https://doi.org/10.1016/j.revpalbo.2017.06.004, 2017.
Ma, Y., Liu, K.-B., Feng, Z., Sang, Y., Wang, W., and Sun, A.: A survey of modern pollen and vegetation along a south–north transect in Mongolia, J. Biogeogr., 35, 1512–1532, https://doi.org/10.1111/j.1365-2699.2007.01871.x, 2008.
Marlon, J. R., Bartlein, P. J., Daniau, A. L., Harrison, S. P., Maezumi, S. Y., Power, M. J., Tinner, W., and Vanniére, B.: Global biomass burning: A synthesis and review of Holocene paleofire records and their controls, Quaternary Sci. Rev., 65, 5–25, https://doi.org/10.1016/j.quascirev.2012.11.029, 2013.
Martin Calvo, M., Prentice, I. C., and Harrison, S. P.: Climate versus carbon dioxide controls on biomass burning: a model analysis of the glacial–interglacial contrast, Biogeosciences, 11, 6017–6027, https://doi.org/10.5194/bg-11-6017-2014, 2014.
Marynowski, L., Bucha, M., Smolarek, J., Wendorff, M., and Simoneit, B. R. T.: Occurrence and significance of mono-, di- and anhydrosaccharide biomolecules in Mesozoic and Cenozoic lignites and fossil wood, Org. Geochem., 116, 13–22, https://doi.org/10.1016/j.orggeochem.2017.11.008, 2018.
Melles, M., Minyuk, P., and Brigham-Grette, J.: The expedition El'gygytgyn Lake 2003 (Siberian Arctic), Berichte zur Polar- und Meeresforschung (Reports on Polar and Marine Research), Bremerhaven, Alfred Wegener Institute for Polar and Marine Research, 509, 139 pp., https://doi.org/10.2312/BzPM_0509_2005, 2005.
Melles, M., Brigham-Grette, J., Glushkova, O. Y., Minyuk, P. S., Nowaczyk, N. R., and Hubberten, H.-W.: Sedimentary geochemistry of core PG1351 from Lake El'gygytgyn–a sensitive record of climate variability in the East Siberian Arctic during the past three glacial–interglacial cycles, J. Paleolimnol., 37, 89–104, https://doi.org/10.1007/s10933-006-9025-6, 2007.
Melles, M., Brigham-Grette, J., Minyuk, P., Koeberl, C., Andreev, A., Cook, T., Fedorov, G., Gebhardt, C., Haltia-Hovi, E., Kukkonen, M., Nowaczyk, N., Schwamborn, G., Wennrich, B., and the El'gygytgyn Scientific Party: The Lake El'gygytgyn Scientific Drilling Project – Conquering Arctic Challenges through Continental Drilling, Sci. Dril., 11, 29–40, https://doi.org/10.2204/iodp.sd.11.03.2011, 2011.
Melles, M., Brigham-Grette, J., Minyuk, P. S., Nowaczyk, N. R., Wennrich, V., DeConto, R. M., Anderson, P. M., Andreev, A. A., Coletti, A., Cook, T. L., Haltia-Hovi, E., Kukkonen, M., Lozhkin, A. V., Rosén, P., Tarasov, P., Vogel, H., and Wagner, B.: 2.8 Million Years of Arctic Climate Change from Lake El'gygytgyn, NE Russia, Science, 337, 315–320, https://doi.org/10.1126/science.1222135, 2012.
Mock, C. J., Bartlein, P. J., and Anderson, P. M.: Atmospheric circulation patterns and spatial climatic variations in Beringia, Int. J. Climatol., 18, 1085–1104, https://doi.org/10.1002/(SICI)1097-0088(199808)18:10<1085::AID-JOC305>3.0.CO;2-K, 1998.
Molinari, C., Lehsten, V., Blarquez, O., Carcaillet, C., Davis, B. A. S., Kaplan, J. O., Clear, J., and Bradshaw, R. H. W.: The climate, the fuel and the land use: Long-term regional variability of biomass burning in boreal forests, Glob. Change Biol., 24, 4929–4945, https://doi.org/10.1111/gcb.14380, 2018.
Nitze, I., Grosse, G., Jones, B. M., Romanovsky, V. E., and Boike, J.: Remote sensing quantifies widespread abundance of permafrost region disturbances across the Arctic and Subarctic, Nat. Commun., 9, 5423, https://doi.org/10.1038/s41467-018-07663-3, 2018.
Nolan, M. and Brigham-Grette, J.: Basic hydrology, limnology, and meteorology of modern Lake El'gygytgyn, Siberia, J. Paleolimnol., 37, 17–35, https://doi.org/10.1007/s10933-006-9020-y, 2007.
Nolan, M., Liston, G., Prokein, P., Brigham-Grette, J., Sharpton, V. L., and Huntzinger, R.: Analysis of lake ice dynamics and morphology on Lake El'gygytgyn, NE Siberia, using synthetic aperture radar (SAR) and Landsat, J. Geophys. Res.-Atmos., 107, ALT 3-1–ALT 3-12, https://doi.org/10.1029/2001jd000934, 2002.
Norwood, M. J., Louchouarn, P., Kuo, L. J., and Harvey, O. R.: Characterization and biodegradation of water-soluble biomarkers and organic carbon extracted from low temperature chars, Org. Geochem., 56, 111–119, https://doi.org/10.1016/j.orggeochem.2012.12.008, 2013.
Nowaczyk, N. R., Melles, M., and Minyuk, P.: A revised age model for core PG1351 from Lake El'gygytgyn, Chukotka, based on magnetic susceptibility variations tuned to northern hemisphere insolation variations, J. Paleolimnol., 37, 65–76, https://doi.org/10.1007/s10933-006-9023-8, 2007.
Nowaczyk, N. R., Haltia, E. M., Ulbricht, D., Wennrich, V., Sauerbrey, M. A., Rosén, P., Vogel, H., Francke, A., Meyer-Jacob, C., Andreev, A. A., and Lozhkin, A. V.: Chronology of Lake El'gygytgyn sediments – a combined magnetostratigraphic, palaeoclimatic and orbital tuning study based on multi-parameter analyses, Clim. Past, 9, 2413–2432, https://doi.org/10.5194/cp-9-2413-2013, 2013.
Oros, D. R. and Simoneit, B. R. T.: Identification and emission factors of molecular tracers in organic aerosols from biomass burning Part 2. Deciduous trees, Appl. Geochem., 16, 1545–1565, https://doi.org/10.1016/S0883-2927(01)00022-1, 2001a.
Oros, D. R. and Simoneit, B. R. T.: Identification and emission factors of molecular tracers in organic aerosols from biomass burning Part 1. Temperate climate conifers, Appl. Geochem., 16, 1513–1544, https://doi.org/10.1016/S0883-2927(01)00021-X, 2001b.
Oros, D. R., Abas, M. R. B., Omar, N. Y. M. J., Rahman, N. A., and Simoneit, B. R. T.: Identification and emission factors of molecular tracers in organic aerosols from biomass burning: Part 3. Grasses, Appl. Geochem., 21, 919–940, https://doi.org/10.1016/j.apgeochem.2006.01.008, 2006.
Otto, A., Gondokusumo, R., and Simpson, M. J.: Characterization and quantification of biomarkers from biomass burning at a recent wildfire site in Northern Alberta, Canada, Appl. Geochem., 21, 166–183, https://doi.org/10.1016/j.apgeochem.2005.09.007, 2006.
Pastorova, I., Arisz, P. W., and Boon, J. J.: Preservation of d-glucose-oligosaccharides in cellulose chars, Carbohyd. Res., 248, 151–165, https://doi.org/10.1016/0008-6215(93)84123-N, 1993.
Pausas, J. G., Keeley, J. E., and Schwilk, D. W.: Flammability as an ecological and evolutionary driver, J. Ecol., 105, 289–297, https://doi.org/10.1111/1365-2745.12691, 2017.
Peters, M. E. and Higuera, P. E.: Quantifying the source area of macroscopic charcoal with a particle dispersal model, Quaternary Res., 67, 304–310, https://doi.org/10.1016/j.yqres.2006.10.004, 2007.
Prentice, C., Guiot, J., Huntley, B., Jolly, D., and Cheddadi, R.: Reconstructing biomes from palaeoecological data: a general method and its application to European pollen data at 0 and 6 ka, Clim. Dynam., 12, 185–194, https://doi.org/10.1007/bf00211617, 1996.
Remy, C. C., Hély, C., Blarquez, O., Magnan, G., Bergeron, Y., Lavoie, M., and Ali, A. A.: Different regional climatic drivers of Holocene large wildfires in boreal forests of northeastern America, Environ. Res. Lett., 12, 035005, https://doi.org/10.1088/1748-9326/aa5aff, 2017.
Revelle, W.: psych: Procedures for Personality and Psychological Research, available at: https://CRAN.R-project.org/package=psych (last access: 21 January 2020), Northwestern University, Evanston, Illinois, USA, 2018.
Rivera-Hernandez, F., Sumner, D. Y., Mackey, T. J., Hawes, I., and Andersen, D. T.: In a PICL: The sedimentary deposits and facies of perennially ice-covered lakes, Sedimentology, 66, 917–939, https://doi.org/10.1111/sed.12522, 2019.
Roberts, A., Roberts, E., and Haigler, C.: Moss cell walls: structure and biosynthesis, Front. Plant Sci., 3, 166, https://doi.org/10.3389/fpls.2012.00166, 2012.
Rogers, B. M., Soja, A. J., Goulden, M. L., and Randerson, J. T.: Influence of tree species on continental differences in boreal fires and climate feedbacks, Nat. Geosci., 8, 228–234, https://doi.org/10.1038/ngeo2352, 2015.
Rolph, G., Stein, A., and Stunder, B.: Real-time Environmental Applications and Display sYstem: READY, Environ. Model. Softw., 95, 210–228, https://doi.org/10.1016/j.envsoft.2017.06.025, 2017.
Sang, X. F., Gensch, I., Kammer, B., Khan, A., Kleist, E., Laumer, W., Schlag, P., Schmitt, S. H., Wildt, J., Zhao, R., Mungall, E. L., Abbatt, J. P. D., and Kiendler-Scharr, A.: Chemical stability of levoglucosan: An isotopic perspective, Geophys. Res. Lett., 43, 5419–5424, https://doi.org/10.1002/2016GL069179, 2016.
Scheffer, M., Hirota, M., Holmgren, M., Van Nes, E. H., and Chapin, F. S.: Thresholds for boreal biome transitions, P. Natl. Acad. Sci. USA, 109, 21384–21389, https://doi.org/10.1073/pnas.1219844110, 2012.
Schmidl, C., Marr, I. L., Caseiro, A., Kotianová, P., Berner, A., Bauer, H., Kasper-Giebl, A., and Puxbaum, H.: Chemical characterisation of fine particle emissions from wood stove combustion of common woods growing in mid-European Alpine regions, Atmos. Environ., 42, 126–141, https://doi.org/10.1016/j.atmosenv.2007.09.028, 2008.
Schreuder, L. T., Hopmans, E. C., Stuut, J.-B. W., Sinninghe Damsté, J. S., and Schouten, S.: Transport and deposition of the fire biomarker levoglucosan across the tropical North Atlantic Ocean, Geochim. Cosmochim. Ac., 227, 171–185, https://doi.org/10.1016/j.gca.2018.02.020, 2018.
Schreuder, L. T., Donders, T. H., Mets, A., Hopmans, E. C., Sinninghe Damsté, J. S., and Schouten, S.: Comparison of organic and palynological proxies for biomass burning and vegetation in a lacustrine sediment record (Lake Allom, Fraser Island, Australia), Org. Geochem., 133, 10–19, https://doi.org/10.1016/j.orggeochem.2019.03.002, 2019.
Schüpbach, S., Kirchgeorg, T., Colombaroli, D., Beffa, G., Radaelli, M., Kehrwald, N. M., and Barbante, C.: Combining charcoal sediment and molecular markers to infer a Holocene fire history in the Maya Lowlands of Petén, Guatemala, Quaternary Sci. Rev., 115, 123–131, https://doi.org/10.1016/j.quascirev.2015.03.004, 2015.
Schuur, E. A. G., McGuire, A. D., Schädel, C., Grosse, G., Harden, J. W., Hayes, D. J., Hugelius, G., Koven, C. D., Kuhry, P., Lawrence, D. M., Natali, S. M., Olefeldt, D., Romanovsky, V. E., Schaefer, K., Turetsky, M. R., Treat, C. C., and Vonk, J. E.: Climate change and the permafrost carbon feedback, Nature, 520, 171–179, https://doi.org/10.1038/nature14338, 2015.
Schwamborn, G., Meyer, H., Fedorov, G., Schirrmeister, L., and Hubberten, H.-W.: Ground ice and slope sediments archiving late Quaternary paleoenvironment and paleoclimate signals at the margins of El'gygytgyn Impact Crater, NE Siberia, Quaternary Res., 66, 259–272, https://doi.org/10.1016/j.yqres.2006.06.007, 2006.
Serreze, M. C. and Barry, R. G.: Processes and impacts of Arctic amplification: A research synthesis, Global Planet. Change, 77, 85–96, https://doi.org/10.1016/j.gloplacha.2011.03.004, 2011.
Simoneit, B. R. T.: Biomass burning – a review of organic tracers for smoke from incomplete combustion, Appl. Geochem., 17, 129–162, https://doi.org/10.1016/S0883-2927(01)00061-0, 2002.
Simoneit, B. R. T., Schauer, J. J., Nolte, C. G., Oros, D. R., Elias, V. O., Fraser, M. P., Rogge, W. F., and Cass, G. R.: Levoglucosan, a tracer for cellulose in biomass burning and atmospheric particles, Atmos. Environ., 33, 173–182, https://doi.org/10.1016/S1352-2310(98)00145-9, 1999.
Sofronov, M. A. and Volokitina, A. V.: Wildfire Ecology in Continuous Permafrost Zone, in: Permafrost Ecosystems: Siberian Larch Forests, edited by: Osawa, A., Zyryanova, O. A., Matsuura, Y., Kajimoto, T., and Wein, R. W., Springer Netherlands, Dordrecht, 59–82, 2010.
Sofronov, M. A., Volokitina, A. V., Kajimoto, T., and Uemura, S.: The Ecological Role of Moss-Lichen Cover and Thermal Amelioration of Larch Forest Ecosystems in the Northern Part of Siberia, Eurasian Journal of Forest Research, 7, 11–19, 2004.
Soja, A. J., Tchebakova, N. M., French, N. H. F., Flannigan, M. D., Shugart, H. H., Stocks, B. J., Sukhinin, A. I., Parfenova, E. I., Chapin, F. S., and Stackhouse, P. W.: Climate-induced boreal forest change: Predictions versus current observations, Global Planet. Change, 56, 274–296, https://doi.org/10.1016/j.gloplacha.2006.07.028, 2007.
Stein, A. F., Draxler, R. R., Rolph, G. D., Stunder, B. J. B., Cohen, M. D., and Ngan, F.: NOAA's HYSPLIT Atmospheric Transport and Dispersion Modeling System, B. Am. Meteorol. Soc., 96, 2059–2077, https://doi.org/10.1175/bams-d-14-00110.1, 2015.
Stivrins, N., Aakala, T., Ilvonen, L., Pasanen, L., Kuuluvainen, T., Vasander, H., Gałka, M., Disbrey, H. R., Liepins, J., Holmström, L., and Seppä, H.: Integrating fire-scar, charcoal and fungal spore data to study fire events in the boreal forest of northern Europe, Holocene, 29, 1480–1490, https://doi.org/10.1177/0959683619854524, 2019.
Suciu, L. G., Masiello, C. A., and Griffin, R. J.: Anhydrosugars as tracers in the Earth system, Biogeochemistry, 146, 209–256, https://doi.org/10.1007/s10533-019-00622-0, 2019.
Sugita, S.: Theory of quantitative reconstruction of vegetation I: pollen from large sites REVEALS regional vegetation composition, Holocene, 17, 229–241, https://doi.org/10.1177/0959683607075837, 2007.
Tarasov, P. E., Andreev, A. A., Anderson, P. M., Lozhkin, A. V., Leipe, C., Haltia, E., Nowaczyk, N. R., Wennrich, V., Brigham-Grette, J., and Melles, M.: A pollen-based biome reconstruction over the last 3.562 million years in the Far East Russian Arctic – new insights into climate–vegetation relationships at the regional scale, Clim. Past, 9, 2759–2775, https://doi.org/10.5194/cp-9-2759-2013, 2013.
Tautenhahn, S., Lichstein, J. W., Jung, M., Kattge, J., Bohlman, S. A., Heilmeier, H., Prokushkin, A., Kahl, A., and Wirth, C.: Dispersal limitation drives successional pathways in Central Siberian forests under current and intensified fire regimes, Glob. Change Biol., 22, 2178–2197, https://doi.org/10.1111/gcb.13181, 2016.
Tchebakova, N. M., Parfenova, E., and Soja, A. J.: The effects of climate, permafrost and fire on vegetation change in Siberia in a changing climate, Environ. Res. Lett., 4, 045013, https://doi.org/10.1088/1748-9326/4/4/045013, 2009.
Theuerkauf, M. and Couwenberg, J.: ROPES Reveals Past Land Cover and PPEs From Single Pollen Records, Front. Earth Sci., 6, 14, https://doi.org/10.3389/feart.2018.00014, 2018.
Thonicke, K., Prentice, I. C., and Hewitt, C.: Modeling glacial-interglacial changes in global fire regimes and trace gas emissions, Global Biogeochem. Cy., 19, GB3008, https://doi.org/10.1029/2004gb002278, 2005.
Tobo, Y., DeMott, P. J., Raddatz, M., Niedermeier, D., Hartmann, S., Kreidenweis, S. M., Stratmann, F., and Wex, H.: Impacts of chemical reactivity on ice nucleation of kaolinite particles: A case study of levoglucosan and sulfuric acid, Geophys. Res. Lett., 39, L19803, https://doi.org/10.1029/2012gl053007, 2012.
van der Werf, G. R., Randerson, J. T., Giglio, L., van Leeuwen, T. T., Chen, Y., Rogers, B. M., Mu, M., van Marle, M. J. E., Morton, D. C., Collatz, G. J., Yokelson, R. J., and Kasibhatla, P. S.: Global fire emissions estimates during 1997–2016, Earth Syst. Sci. Data, 9, 697–720, https://doi.org/10.5194/essd-9-697-2017, 2017.
van Leeuwen, T. T. and van der Werf, G. R.: Spatial and temporal variability in the ratio of trace gases emitted from biomass burning, Atmos. Chem. Phys., 11, 3611–3629, https://doi.org/10.5194/acp-11-3611-2011, 2011.
Veraverbeke, S., Rogers, B. M., Goulden, M. L., Jandt, R. R., Miller, C. E., Wiggins, E. B., and Randerson, J. T.: Lightning as a major driver of recent large fire years in North American boreal forests, Nat. Clim. Change, 7, 529, 529–534, https://doi.org/10.1038/nclimate3329, 2017.
Wasserstein, R. L., Schirm, A. L., and Lazar, N. A.: Moving to a World Beyond “p < 0.05”, Am. Stat., 73, 1–19, https://doi.org/10.1080/00031305.2019.1583913, 2019.
Wennrich, V., Francke, A., Dehnert, A., Juschus, O., Leipe, T., Vogt, C., Brigham-Grette, J., Minyuk, P. S., Melles, M., and El'gygytgyn Science Party: Modern sedimentation patterns in Lake El'gygytgyn, NE Russia, derived from surface sediment and inlet streams samples, Clim. Past, 9, 135–148, https://doi.org/10.5194/cp-9-135-2013, 2013.
Wennrich, V., Andreev, A. A., Tarasov, P. E., Fedorov, G., Zhao, W., Gebhardt, C. A., Meyer-Jacob, C., Snyder, J. A., Nowaczyk, N. R., Schwamborn, G., Chapligin, B., Anderson, P. M., Lozhkin, A. V., Minyuk, P. S., Koeberl, C., and Melles, M.: Impact processes, permafrost dynamics, and climate and environmental variability in the terrestrial Arctic as inferred from the unique 3.6 Myr record of Lake El'gygytgyn, Far East Russia – A review, Quaternary Sci. Rev., 147, 221–244, https://doi.org/10.1016/j.quascirev.2016.03.019, 2016.
Westerling, A. L., Hidalgo, H. G., Cayan, D. R., and Swetnam, T. W.: Warming and Earlier Spring Increase Western U.S. Forest Wildfire Activity, Science, 313, 940–943, https://doi.org/10.1126/science.1128834, 2006.
Whitlock, C. and Larsen, C.: Charcoal as a Fire Proxy, in: Tracking Environmental Change Using Lake Sediments. Terrestrial, Algal, and Siliceous Indicators, edited by: Smol, J. P., Birks, H. J. B., and Last, W. M., KluwerAcademic Publishers, Dordrecht, the Netherlands, 75–97, 2001.
Williams, R. S. J. and Ferrigno, J. G.: State of the Earth's cryosphere at the beginning of the 21st century–Glaciers, global snow cover, floating ice, and permafrost and periglacial environments, U.S. Geological Survey Professional Paper, 1386–A, edited by: USGS, United States Government Printing Office, Washington, 546 pp., 2012.
Wirth, C.: Fire Regime and Tree Diversity in Boreal Forests: Implications for the Carbon Cycle, Ecological Studies: Forest Diversity and Function: Temperate and Boreal Systems edited by: Scherer-Lorenzen, M., Körner, C., and Schulze, E. D., Springer-Verlag, Berlin, Heidelberg, 2005.
Yang, H., Yan, R., Chen, H., Lee, D. H., and Zheng, C.: Characteristics of hemicellulose, cellulose and lignin pyrolysis, Fuel, 86, 1781–1788, https://doi.org/10.1016/j.fuel.2006.12.013, 2007.
Yin, Q. and Berger, A.: Interglacial analogues of the Holocene and its natural near future, Quaternary Sci. Rev., 120, 28–46, https://doi.org/10.1016/j.quascirev.2015.04.008, 2015.
Yin, Q. Z. and Berger, A.: Individual contribution of insolation and CO2 to the interglacial climates of the past 800,000 years, Clim. Dynam., 38, 709–724, https://doi.org/10.1007/s00382-011-1013-5, 2012.
Zhang, N., Yasunari, T., and Ohta, T.: Dynamics of the larch taiga–permafrost coupled system in Siberia under climate change, Environ. Res. Lett., 6, 024003, https://doi.org/10.1088/1748-9326/6/2/024003, 2011.
Zhao, W., Andreev, A. A., Tarasov, P. E., Wennrich, V., and Melles, M.: Vegetation and climate during the penultimate interglacial of the northeastern Russian Arctic: the Lake El'gygytgyn pollen record, Boreas, 48, 507–515, https://doi.org/10.1111/bor.12373, 2019.